
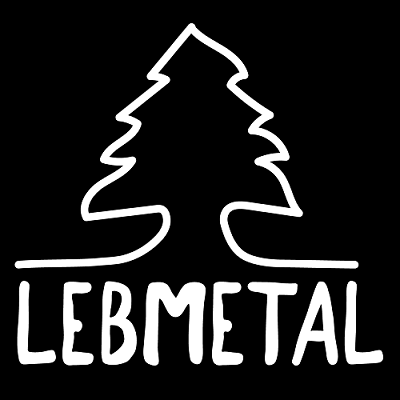

Heavy Metal and Masaru Emoto’s Water Crystals
Contributed to this article.
“Masaru Emoto was a Japanese author, researcher and entrepreneur, who claimed that human consciousness has an effect on the molecular structure of water.” – Wikipedia
It was 1994 when the idea to freeze water and observe it with microscope came upon me. With this method, I was convinced that I should be able to see something like snow crystals. … from the water from rivers and lakes where water is kept pristine from development, we could observe beautiful crystals with each one having its own uniqueness. – masaru-emoto.net
The observation was done in various ways:
> Showing letters to water
> Showing pictures to water
> Playing music to water
> Praying to water
Here are some observations of frozen water after being exposed to different music genres:
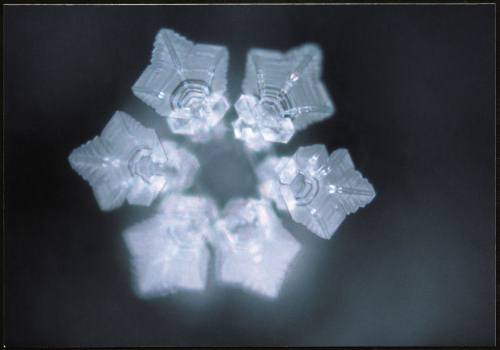
John Lennon – Imagine – masaru-emoto.net
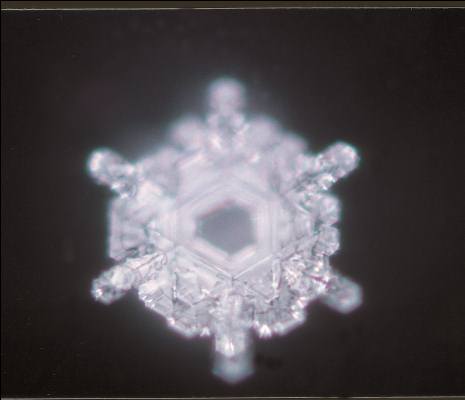
The Beatles – Yesterday – masaru-emoto.net
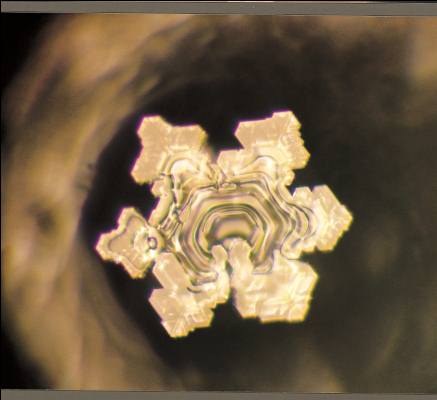
Mozart – Symphony No. 40 – masaru-emoto.net
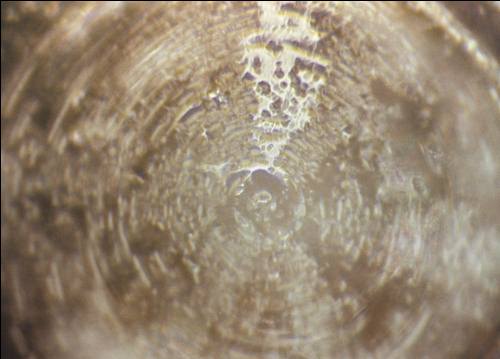
A Heavy Metal Song – masaru-emoto.net
While most music genres used in these experiments positively influence water and create such beautiful designs, Heavy Metal simply seems to put the water’s “inner energy” into a deep well of basically … shit. It is worthwhile noting that the “Showing letters to water” experiments had a similar destructive output when showing the words “Evil” to water:
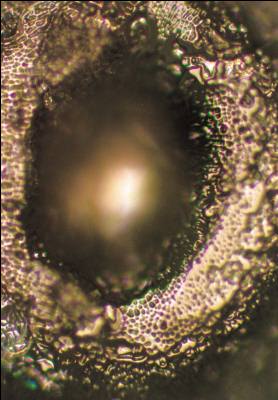
Water after being exposed to the word “Evil” – masaru-emoto.net
The full experiments photos can be viewed here: https://www.masaru-emoto.net/en/science-of-messages-from-water
If I would be showing these results to a fellow Metalhead, there’s a 90% chance that our first reaction would be in the form of James Hetfield’s Yeaaaaaaaa .

Copyrights LebMetal 2009-2023
Privacy Policy
A review of mesocosm experiments on heavy metals in marine environment and related issues of emerging concerns
Affiliations.
- 1 National Centre for Coastal Research, Ministry of Earth Sciences, Government of India, NIOT Campus, Pallikaranai, Chennai, 600 100, India.
- 2 National Centre for Coastal Research, Ministry of Earth Sciences, Government of India, NIOT Campus, Pallikaranai, Chennai, 600 100, India. [email protected].
- PMID: 33079346
- DOI: 10.1007/s11356-020-11121-3
Mesocosms are real-world environmental science tools for bridging the gap between laboratory-scale experiments and actual habitat studies on ecosystem complexities. These experiments are increasingly being applied in understanding the complex impacts of heavy metals, ocean acidification, global warming, and oil spills. The insights of the present review indicate how metals and metal-bound activities impact on various aspects of ecological complexities like prey predator cues, growth, embryonic development, and reproduction. Plankton and benthos are used more often over fish and microbes owing to their smaller size, faster reproduction, amenability, and repeatability during mesocosm experiments. The results of ocean acidification reveal calcification of plankton, corals, alteration of pelagic structures, and plankton blooms. The subtle effect of oil spills is amplified on sediment microorganisms, primary producers, and crustaceans. An overview of the mesocosm designs over the years indicates that gradual changes have evolved in the type, size, design, composition, parameters, methodology employed, and the outputs obtained. Most of the pelagic and benthic mesocosm designs involve consideration of interactions within the water columns, between water and sediments, trophic levels, and nutrient rivalry. Mesocosm structures are built considering physical processes (tidal currents, turbulence, inner cycling of nutrients, thermal stratification, and mixing), biological complexities (population, community, and ecosystem) using appropriate filling containers, and sampling facilities that employ inert materials. The principle of design is easy transportation, mooring, deployment, and free floating structures besides addressing the unique ecosystem-based science problems. The evolution of the mesocosm tools helps in understanding further advancement of techniques and their applications in marine ecosystems.
Keywords: Ecosystem; Marine pollution; Mesocosm; Natural hazards; Sustainable management.
Publication types
- Hydrogen-Ion Concentration
- Metals, Heavy*
- Metals, Heavy
Grants and funding
- MoES/EFC/28/2018-PC-II dated 12/11/2018/Ministry of Earth Sciences
Academia.edu no longer supports Internet Explorer.
To browse Academia.edu and the wider internet faster and more securely, please take a few seconds to upgrade your browser .
Enter the email address you signed up with and we'll email you a reset link.
- We're Hiring!
- Help Center
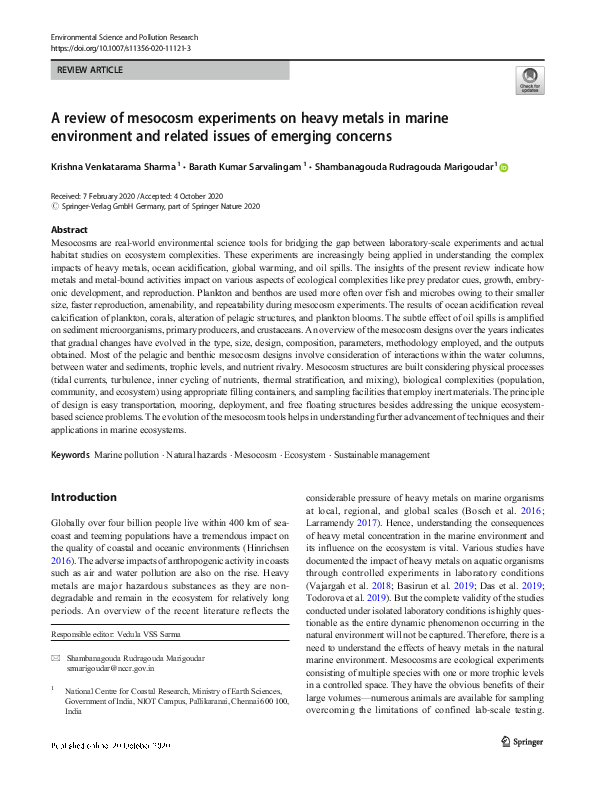
Download Free PDF
A review of mesocosm experiments on heavy metals in marine environment and related issues of emerging concerns

Mesocosms are real-world environmental science tools for bridging the gap between laboratory-scale experiments and actual habitat studies on ecosystem complexities. These experiments are increasingly being applied in understanding the complex impacts of heavy metals, ocean acidification, global warming, and oil spills. The insights of the present review indicate how metals and metal-bound activities impact on various aspects of ecological complexities like prey predator cues, growth, embryonic development, and reproduction. Plankton and benthos are used more often over fish and microbes owing to their smaller size, faster reproduction, amenability, and repeatability during mesocosm experiments. The results of ocean acidification reveal calcification of plankton, corals, alteration of pelagic structures, and plankton blooms. The subtle effect of oil spills is amplified on sediment microorganisms, primary producers, and crustaceans. An overview of the mesocosm designs over the years indicates that gradual changes have evolved in the type, size, design, composition, parameters, methodology employed, and the outputs obtained. Most of the pelagic and benthic mesocosm designs involve consideration of interactions within the water columns, between water and sediments, trophic levels, and nutrient rivalry. Mesocosm structures are built considering physical processes (tidal currents, turbulence, inner cycling of nutrients, thermal stratification, and mixing), biological complexities (population, community, and ecosystem) using appropriate filling containers, and sampling facilities that employ inert materials. The principle of design is easy transportation, mooring, deployment, and free floating structures besides addressing the unique ecosystembased science problems. The evolution of the mesocosm tools helps in understanding further advancement of techniques and their applications in marine ecosystems.
Related papers
Frontiers in Ecology and the Environment, 2016
Marine ecologists have a wide array of tools with which to study complex and dynamic systems, but there are cases where neither simple, highly controlled experiments nor largely uncontrolled, more complex field observations provide adequate inferential power. In such cases, mesocosm studies in marine systems may help bridge the gap. Mesocosm studies can facilitate research ranging from basic biology to multifactorial ecosystem studies that involve observation, perturbation, validation, calibration, long-term studies, and testing of new technologies. Although scale, closed boundaries, biodiversity levels, and replication can impose challenges on mesocosm research, these parameters can also help to define research opportunities that are uniquely suited to such controlled environments. Finally, we provide examples of successful marine mesocosm research and discuss opportunities for future work.
Ecology and Evolution, 2015
We describe a completely randomizable flow-through outdoor mesocosm for climate change and ecotoxicology studies that was built with inexpensive materials. The 16 raceway tanks allow up to 69 water renewal per hour, avoiding changes in natural abiotic seawater conditions. We use an open-source hardware board (Arduino) that was adapted to control heaters and an innovative CO 2 injection system. This system reduced seawater pH up to À0.9 units and increased temperature up to +6°C in three treatments and a control. Treatments can be continuously compared with the control and vary according to diel fluctuations, thus following the diel range observed in the sea. The mesocosm facility also includes an integrated secondary system of 48 aquaria for ecotoxicology studies. We validated the reproducibility and relevance of our experimental system by analyzing the variation of the total DNA of the microbial community extracted from corals in three elevated temperature scenarios during a 40-day experiment. We also present data from temperature, acidification, and copper contamination trials, which allowed continuous, reliable, and consistent treatment manipulations.
Limnology and Oceanography-methods, 2017
A new mesocosm setup containing both water column and sediment suitable for benthic-pelagic coupling experiments is described. The presence of a large volume of water (>1.5 m 3) with sufficient depth (4 m) on the top of the sediment, combined with a large volume of sediment on the bottom (30 cm height, 80 L volume) offers new opportunities for benthic-pelagic experiments on the mesocosm scale. The experimental setup includes a mesocosm bag, a securing ring with a cap on the top, a sediment container on the bottom, sediment traps, an autonomous water sampling system at specific depths, and a newly developed sediment sampler for collecting samples without disturbing the system. The mesocosm setup was successfully deployed in the context of a benthic-pelagic coupling eutrophication experiment in the CRETACOSMOS mesocosm facilities of the Hellenic Centre for Marine Research in Crete. A number of variables related to the technical aspects and proper ecosystem functions of the mesocosm setup were monitored throughout the duration of the experiment (58 d), which proved that the proposed setup is suitable for benthic-pelagic coupling experiments, providing an intermediate experimental tool between small-scale micro/benthocosm experiments and large scale in situ sea experiments.
There is now widespread recognition that chemical monitoring of the environment is not suficient given that pollution is essentially a biological phenornenon because of its impact on living organisms. Careful interpretation of the biota, in light of the known ecology of the species involved, is needed to ascertain whether there is impact, and may even indicate the nature of the cause. An approach developed in Great Britain (RIVPACS) provides a method for biological surveillance, conservation, and environmental impact assessment in rivers. It predicts the macroinvertebrate fauna to be expected at a given site based on a small number of environmental features. Using the mode!, it is possible to predict the benthic community that should occur at a site. A modified approach to that described in the British Riven study has been used in the assessment of the Great Lakes. A goal of that study was to predict what the benthic community should look like at a site if it were undisturbed. The a...
Limnetica, 2003
Much of the past research in aquatic ecology has been based on data collected in uncontrolled situations in the field. Indeed, the results of in vitro experiments cannot easily be extrapolated to complex natural ecosystems. On the other hand, the whole-lake experiments (Schindler, 1975)
Anthropogenic carbon dioxide (CO 2) emissions are causing severe changes in the global inorganic carbon balance of the oceans. Associated ocean acidification is expected to impose a major threat to marine ecosystems worldwide, and it is also expected to be amplified in the Baltic Sea where the system is already at present exposed to relatively large natural seasonal and diel pH fluctuations. The response of organisms to future ocean acidification has primarily been studied in single-species experiments, whereas the knowledge of community-wide responses is still limited. To study responses of the Baltic Sea pelagic community to a range of future CO 2-scenarios, six ∼ 55 m 3 pelagic mesocosms were deployed in the northern Baltic Sea in June 2012. In this specific study we focused on the tolerance, development and subsequent settlement process of the larvae of the benthic key-species Macoma balthica when exposed to different levels of future CO 2. We found that the settling of M. balthica was delayed along the increasing CO 2 gradient of the mesocosms. Also, when exposed to increasing CO 2 levels larvae settled at a larger size, indicating a developmental delay. With ongoing climate change, both the frequency and extent of regularly occurring high CO 2 conditions is likely to increase, and a permanent pH decrease will likely occur. The strong impact of increasing CO 2 levels on early-stage bivalves is alarming as these stages are crucial for sustaining viable populations, and a failure in their recruitment would ultimately lead to negative effects on the population.
Ecology and Evolution
This is an open access article under the terms of the Creative Commons Attribution License, which permits use, distribution and reproduction in any medium, provided the original work is properly cited.
Horado 1751·1101, Mhico lI~HOt D. F. Esta edición y sus características son propiedad de la EDITORIAL PORRÚA. S. A. DE C. V.-2 Av. República Argentina, 15,06020, México, D. F. ISBN 970-07-5786-2 lMPRLSO EN MtXICO PRINTED IN MEXlCO TEORiA DEL ESTADO 15 nen como finalidad común el máximo respeto a la persona humana y su dignidad.
The Homeless Hub, 2019
ŚWIATOWIT Supplement Series B: Barbaricum vol. 11, 2015
“The Qur’an of Guglielmo Raimondo Moncada: the Arabic Versions of Suras 21 and 22 in BAV MS Urb. Lat. 1384, in The Qur’an in Rome. Manuscripts, Translations, and the Study of Islam in Early Modern Catholicism, edited by F. Stella and R. Tottoli, Berlin – Boston, De Gruyter, 55-78, 2024
Journal of Trauma Studies in Education, 2023
Annals of Translational Medicine
Forest Ecology and Management, 2008
Revista Brasileira de Historia da Ciencia 15:325-346, 2022
Economic Review, 1999
INNOVA Research Journal, 2017
Geoderma, 1996
BMC Musculoskeletal Disorders, 2008
Jurnal ARTESIS
Medicinski glasnik : official publication of the Medical Association of Zenica-Doboj Canton, Bosnia and Herzegovina, 2021
Acta Crystallographica Section A, 1987
IUBMB Life (International Union of Biochemistry and Molecular Biology: Life), 2002
Comparative Biochemistry and Physiology Part A: Molecular & Integrative Physiology, 2008
Related topics
- We're Hiring!
- Help Center
- Find new research papers in:
- Health Sciences
- Earth Sciences
- Cognitive Science
- Mathematics
- Computer Science
- Academia ©2024
An official website of the United States government
Official websites use .gov A .gov website belongs to an official government organization in the United States.
Secure .gov websites use HTTPS A lock ( Lock Locked padlock icon ) or https:// means you've safely connected to the .gov website. Share sensitive information only on official, secure websites.
- Publications
- Account settings
- Advanced Search
- Journal List

Heavy metal tolerance and accumulation in the Brassica species ( Brassica chinensis var. parachinensis and Brassica rapa L.): A pot experiment
Adzrin asikin zunaidi, lee hoon lim, faizah metali.
- Author information
- Article notes
- Copyright and License information
Corresponding author. [email protected]
Received 2023 Sep 18; Revised 2024 Apr 8; Accepted 2024 Apr 9; Collection date 2024 Apr 30.
This is an open access article under the CC BY-NC license (http://creativecommons.org/licenses/by-nc/4.0/).
This study delves into the heavy metal tolerance and accumulation capabilities of Brassica chinensis var. parachinensis ( B. chinensis ) and Brassica rapa L. ( B. rapa ) in a pot experiment, specifically focusing on cadmium (Cd), chromium (Cr) and lead (Pb). Agricultural topsoils were spiked with varying concentrations of these heavy metals (0 mg/kg, 75 mg/kg, 150 mg/kg, 225 mg/kg and 300 mg/kg) for each element. The experiment involved cultivating 15 pots each of B. chinensis and B. rapa over 60 days. Results indicated that both Brassica species experienced delayed germination, with B. chinensis exhibiting a significant drop in germination percentage to 53 % at the highest concentration (300 mg/kg), while B. rapa showed a tendency for an increased germination percentage of up to 80 % at elevated metal concentrations; however, these differences were not statistically significant. Both B. chinensis and B. rapa demonstrated a stable decline in growth rate from 0.05 cm/day to 0.04 cm/day with increasing heavy metal concentrations, and the he reduction in relative growth rate was significant at the highest concentration compared to the control. The stress tolerance index revealed a significant decrease in plant heights for B. chinensis , in contrast to the stable performance of B. rapa , showcasing the tolerance of B. rapa to toxic conditions. Despite insignificant differences in fresh biomass due to metal treatments, B. chinensis consistently yielded higher biomass, yet it had a lower edible index due to its higher root biomass. Leaf areas increased significantly in both species at higher soil treatments, while root lengths remained unchanged, suggesting their resilience to elevated heavy metal concentrations. Analysis of plant tissues (leaves, stems and roots) using ICP-OES revealed that B. rapa accumulated the highest Cd concentration (864 mg/kg), whereas B. chinensis accumulated the highest Pb concentration (953 mg/kg) in root parts. Both species significantly accumulated Cr in roots, demonstrating a sequestration mechanism. These findings suggest that both species, particularly, B. rapa possess strong tolerance and accumulation capabilities for non-essential heavy metals, making them potential hyperaccumulators for green remediation techniques in toxic soil environments. Understanding the molecular mechanisms driving these responses and validating phytoremediation potential in real-world scenarios is essential for developing sustainable soil management practices.
Keywords: Ecotoxicology, Hyperaccumulators, Metal stress response, Phytoremediation, Plant-metal interactions, Soil pollution
1. Introduction
Heavy metals pose serious risks to plants and humans due to their high toxicity and persistence in natural ecosystems [ [1] , [2] , [3] ]. Their presence in plants can disrupt vital biochemical processes such as nutrient homeostasis, antioxidant and enzyme production, gas exchange components, and photosynthesis [ 4 ]. Various remediation techniques, including phytoremediation, chemical and physical methods, and microbial remediation, have been employed to mitigate heavy metal contamination in soil [ 5 ]. Phytoremediation, recognized as a cost-effective green technique, involves using plants to absorb and accumulate contaminants, such as heavy metals, through their root systems, effectively removing them from the environment [ 6 , 7 ]. Commonly employed phytoremediation procedures include phytodegradation, phytoextraction, phytostabilisation, phytovolatilisation, and rhizofiltration [ 8 ].
In phytoremediation, assessing different plant species' properties is crucial for understanding their tolerance and accumulation abilities against specific contaminants [ 9 ]. Phytotolerance analyses are essential to gauge metal tolerance levels, investigating the negative impacts of heavy metals on morphological, physiological, and biochemical activities in plants [ 10 ]. Monitoring stress tolerance during seed germination and seedling stages is particularly valuable, given the vulnerability of seeds to toxic environments compared to the vegetative stage [ 11 , 12 ]. Evaluating metal stress in seedlings provides insights into their growth performance under toxic conditions. Numerous studies have highlighted the effects of heavy metal-contaminated soils on germination, often resulting in reduced performance or seedling damage in various plant species [ [13] , [14] , [15] , [16] ]. These effects can, in turn, impact physicochemical processes such as enzyme activities and photosynthesis [ 4 , 17 ].
Some recognized phytoaccumulators, like Ariplex halimus L. and Halimione portulacoides Aellen, are deemed suitable for accumulating cadmium (Cd), chromium (Cr), copper (Cu), mercury (Hg) and lead (Pb), contributing to phytostabilization in saline soils [ [18] , [19] , [20] , [21] , [22] ]. These hyperaccumulator plants release protons from their roots, increasing soil acidity and facilitating metal ion mobility, thereby enhancing metal uptake into roots and shoots [ 5 ]. Ideal hyperaccumulating plants exhibit traits such as high heavy metal concentrations, tolerance to heavy metals, adaptability to toxic environments, fast growth, high biomass, and harvestability [ 23 ]. Efficient metal translocation from roots to shoots is also crucial. Currently, only a few plant species, including those from Asteraceae, Brassicaceae, Caryophyllaceae, Cunoniaceae, Cyperaceae, Euphorbiaceae, Fabaceae, Flacourtiaceae, Lamiaceae, Poaceae, and Violaceae, meet these criteria [ [24] , [25] , [26] , [27] ]. Given that different plant species accumulate various contaminants, careful plant selection for phytoextraction, a key phytoremediation method for heavy metals in soils, is essential [ 28 ]. While some studies have explored the use of woody plants like Populus tremula and Picea abies for soil decontamination, their limited extraction and accumulation of heavy metals pose disadvantages, restricting their use for phytoextraction [ [29] , [30] , [31] ].
Several non-woody plants, including Brassica species like Alyssum Bertolonii , Alyssum murale , Noccaeae caerulescens , Streptanthus polygaloides , Stanleya pinnata and Bornmuellera tymphaea , are known as metal accumulators [ 32 ]. The potential application of Brassica species for phytoextraction of heavy metals is mainly due to their underlying tolerance to heavy metals [ 33 ]. Brassica species are valued for their ability to tolerate heavy metals, particularly B. juncea , B. napus , B. oleracea , B. carinata and B. nigra , which have shown high tolerance to toxic metal concentrations in soils [ 34 , 35 ]. Studies have demonstrated the accumulation of metals like Cu, nickel (Ni), Pb, and zinc (Zn) in B. juncea compared to B. campestris , B. carinata , B. napus and B. nigra in a field or pot experiment, indicating its potential for phytoextraction [ 36 ]. B. juncea accumulated Cd, cobalt (Co), Ni, Pb and Zn in leaves, stems and roots, but only Cu and manganese (Mn), as well as Cr, in the stems and roots based on the metal transfer factor of more than 1 [ 37 ]. B. chinensis accumulated higher levels of Cd, Cr and Pb in all plant parts than B. rapa in a pot study using agricultural soils [ 38 ]. Further research is needed to explore metal uptake and tolerance in other Brassica species.
This study aims to determine the tolerance and accumulation rates of two Brassica species ( Brassica chinensis var. parachinensis , B. chinensis hereafter, and Brassica rapa L., B. rapa hereafter) to Cd, Cr, and Pb, selected due to their prevalence in Brunei Darussalam's agricultural soils [ 38 ]. These species were chosen for their common presence in Brunei's farmland and their known high uptake capacities for these metals [ 38 , 39 ]. The study assesses the phytotolerance of B. chinensis and B. rapa to various concentrations of heavy metal contaminants, alongside evaluating their effects on growth performance. The specific objectives are as follows: (1) to monitor the growth of the two Brassica species from seed germination to mature stage at different concentrations of selected heavy metals contaminants (Cd, Cr and Pb), (2) to investigate the heavy metals tolerance levels of B. chinensis and B. rapa , and (3) to assess the phytoremediation potential of using B. chinensis and B. rapa to accumulate heavy metals in soils.
2. Materials and methods
2.1. study area.
The pot experiment with B. chinensis and B. rapa took place within a plant shade (6 m long x 4 m wide x 2 m high) which is located at the Faculty of Science, Universiti Brunei Darussalam, situated in Brunei Darussalam (4°58′31.1″ N, 114°53′48.6″ E) on the north-west side of the Borneo Island in Southeast Asia ( Fig. 1 ). The plant shade was covered with a black shade netting (50 % light transmission) on the top and sides to shield the growing vegetables from direct sunlight, which could impede their early growth and survival. Additionally, a transparent plastic roof was used to cover the top of the plant shade, ensuring that the experiment remained unaffected by rainfall.
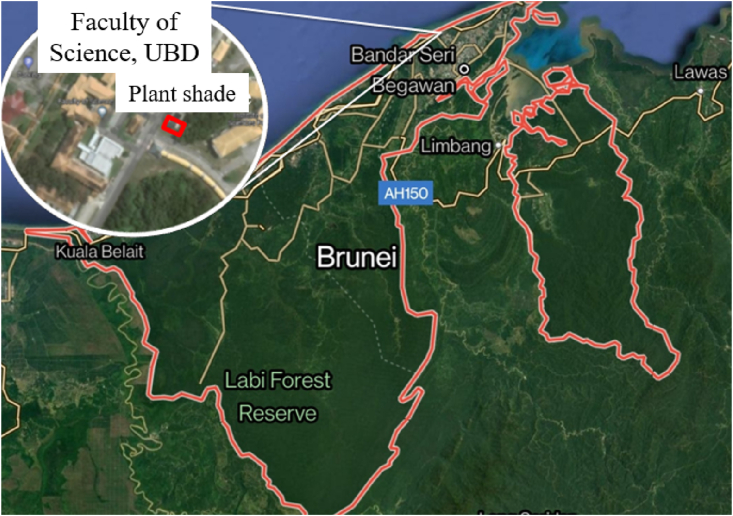
Location of plant shade at the Faculty of Science, Universiti Brunei Darussalam, Brunei Darussalam (4°58′31.1″ N, 114°53′48.6″ E). Source: https://www.google.com/maps ; Accessed 12 th November 2023. (For interpretation of the references to colour in this figure legend, the reader is referred to the Web version of this article.)
Brunei Darussalam experiences a humid equatorial climate. Throughout our study's growth phases from December 2021 to February 2022, the average monthly temperature ranged from 25.6 °C to 28.7 °C, with an average of 27.3 °C, while the average monthly rainfall varied from 2.1 mm to 31 mm, averaging at 8.7 mm (Brunei Darussalam Meteorological Department, unpublished data). Fig. 2 illustrates the average weekly temperature (°C) and rainfall (mm) over an 8-week period of this study.
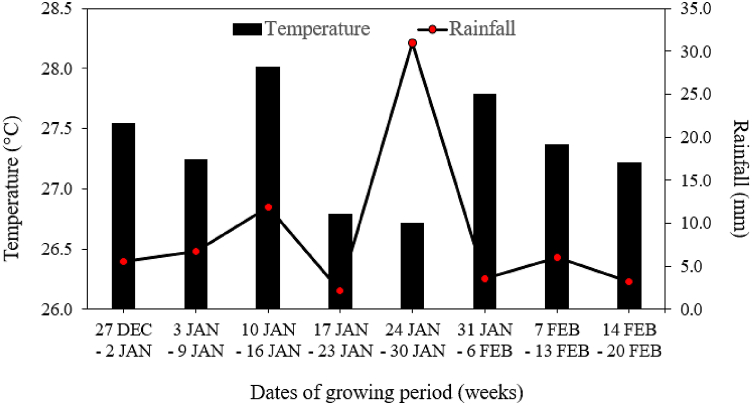
Average weekly temperature (°C) and rainfall (mm) during the growth period of Brassica chinensis var. parachinensis and Brassica rapa L. from December 2021 to February 2022. Data was sourced from the Brunei Darussalam Meteorological Department (BDMD), Ministry of Transport and Infocommunication, Brunei Darussalam, located approximately 4 km from the study site.
2.2. Study species, sampling and sample pre-treatment
The chosen plant species, B. chinensis and B. rapa , are annual herbaceous plants which are known for their potential in soil heavy metal phytoremediation [ 40 ]. These Brassica species are versatile, with edible leaves and stems that can be consumed cooked or raw, and they are also utilized for seed oil production and as green manure [ 35 , 41 ]. Seeds of B. chinensis and B. rapa utilized in this study were sourced from local markets in Brunei Darussalam.
In July 2021, agricultural topsoil (120 kg) was collected from 10 random locations at the Bukit Agok agricultural farmland, located in the Brunei-Muara district of Brunei Darussalam (4°54′58.9″N, 114°47′44.4″E). These soil samples were carefully stored in labeled plastic basins and transported to the laboratory for pre-treatment and analysis. Before any treatment, the soil samples were air-dried and sieved through a 2 mm mesh sieve to remove stone pebbles and plant litter. Following drying, the soil samples ( n = 10) were analyzed for physicochemical properties with triplicate reading. The soil analyses included texture classification [ 42 ], moisture content [ 43 ], pH [ 44 ], electrical conductivity [ 45 ], total organic carbon (OC) and organic matter (OM) content [ 46 ], total N, P, K, Ca, Mg and Na [ 47 ] and cation exchange capacity (CEC) [ 48 ]. The summarized data is presented in Table 1 .
Physicochemical properties of agricultural soils at Bukit Agok, Brunei Darussalam, utilized in the pot experiments for assessing heavy metal tolerance in Brassica species. Values represent the mean with standard deviation (SD) for n = 10.
* Soil classification is based on the World Reference Base for Soil Resources [ 49 ].
In this study, a total of 30 polyethylene pots (22.5 cm diameter × 20.0 cm height) were arranged inside the plant shade. Following soil analysis, the soils were mixed and bulked. About 3 kg of air-dried soil was then distributed into each pot, spiked with uniform concentrations of Cd, Cr, and Pb at four levels: 75 mg/kg (T1), 150 mg/kg (T2), 225 mg/kg (T3), and 300 mg/kg (T4) of Cd (CdCl 2 ), Cr (CrCl 3 .6H 2 O), and Pb (Pb(CH 3 COO) 2 .3H 2 O) as heavy metal pollutants, with triplicates for each treatment. The chosen concentration range is aligned with the maximum allowable limits set by the United States Environmental Protection Agency (USEPA) [ 50 ].
Soil spiking in each pot involved preparing individual concentration for each metal (Cd, Cr and Pb), followed by mixing to create the treatment concentrations. This was achieved by adding precise amounts of Cd, Cr and Pb salts dissolved in distilled water into each pot containing 3 kg of soil. Thorough mixing of the soils was conducted using clean plastic trowels, followed by a 2-week period for drying and stabilization at room temperature. This procedure was based on a modified protocol derived from Mkumbo [ 50 ] and Amin et al. [ 51 ]. Control pots (T0) were also set up with no addition of pollutants. The pot experiment involving varying concentrations of Cd, Cr, and Pb served to systematically explore the responses of B. chinensis and B. rapa to different levels of heavy metal contamination. This approach established a dose-response relationship, allowing researchers to identify threshold levels impacting germination, growth rates, and metal accumulation. By simulating realistic local soil conditions in Bukit Agok, Brunei Darussalam, the experiment enables the assessment of species-specific responses and evaluates the phytoremediation potential of these Brassica species. The controlled environment of the pot experiment enhances the reliability of observations, providing valuable insights into the adaptability and suitability of these plants for sustainable soil management practices.
The pots were arranged in a completely randomized design within the plant shade, resulting in 15 pots (3 pots x 5 treatments) for each species. Each pot was planted with a total of 20 seeds, yielding 60 seeds per treatment for each species. Germination progress for both the Brassica species was observed daily until day 10, with plants receiving irrigation of 100 mL distilled water three times weekly. After germination bioassay, seedlings were thinned to maintain three seedlings per pot, resulting in three B. chinensis or three B. rapa plants per pot. Daily weeding was performed to ensure the unhindered growth of the study species. Both B. chinensis and B. rapa typically germinated within 5 days and reached maturity in approximately 5 weeks ( Fig. 3 A0 – A4 and B0 – B4), following the BBCH scale which was used to assess the principal growth stages of the two species under various soil treatments, they can be described using numbers in ascending order [ 52 ]. In this experiment, no fertilizer was applied to ensure that the spiked soils remained the sole source of pollutants. After 60 days, both species were harvested in February 2022. Plants from each pot were bulked for chemical analysis in triplicates, resulting in three samples per treatment per species.
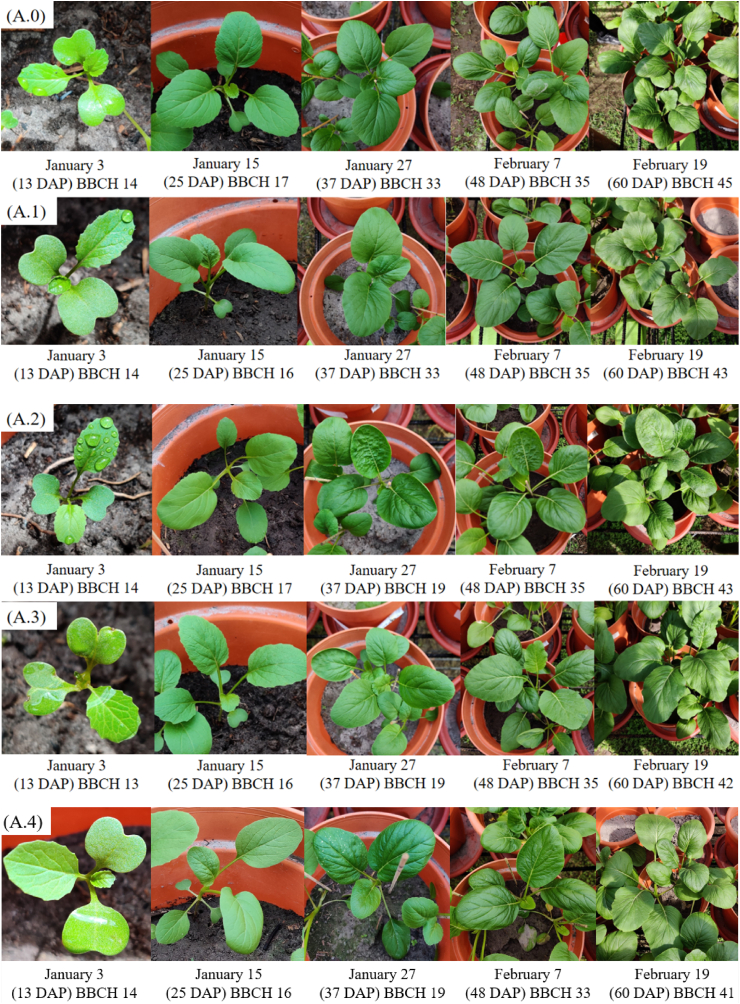
Phenological stages of (A): Brassica chinensis var. parachinensis at different heavy metal treatments at different days after planting (DAP) – A.0: Control or Treatment 0, A.1: Treatment 1, A.2: Treatment 2, A.3: Treatment 3 and A.4: Treatment 4, and (B): Brassica rapa L. at different treatments – B.0: Control, B.1: Treatment 1, B.2: Treatment 2, B.3: Treatment 3 and B.4: Treatment 4, according to the BBCH scale [ 52 ]: 12 (second true leaf unfolded), 13(third true leaf unfolded), 14 (fourth true leaf unfolded), 16 (sixth true leaf unfolded), 17 (seventh true leaf unfolded), 18 (eight true leaf unfolded), 19 (ninth true leaf unfolded), 33 (leaf rosette has reached 30 % of expected diameter), 35 (leaf rosette has reached 50 % of expected diameter), 41 (10 % of the leaf mass typical of the variety reached), 42 (20 % of the leaf mass typical of the variety reached), 43 (30 % of the leaf mass typical of the variety reached), 44 (40 % of the leaf mass typical of the variety reached, 45 (50 % of the leaf mass typical of the variety reached) and 47 (70 % of the leaf mass typical of the variety reached).
2.3. Data analysis
Seven plant growth measurements were conducted for each Brassica vegetable to assess their tolerance levels and ecotoxicological effects under varied soil treatments. These measurements included germination percentage, relative growth rate, stress tolerance index, fresh biomass, edible index, leaf area, and maximum root length.
2.3.1. Germination percentage
The germination percentage (G%) of B. chinensis and B. rapa was determined by calculating the ratio of germinated seeds to the total number of viable seeds sown in each metal-spiked treatment, following Eq. (1) [ 53 ], observed over a 10-day period:
where G and G 0 represent the number of germinated seeds in each treatment and the total number of seeds sowed in each treatment, respectively. In this study, 20 seeds were sown in each pot, resulting in a total of 300 viable seeds and 60 seeds per treatment for each species.
2.3.2. Relative growth rate of species
The relative growth rate (RGR) or efficiency index (EI) of both B. chinensis and B. rapa was assessed by measuring plant height under various metal-spiked soil treatments over a 60-day period, as per Eq. (2) [ 54 ]:
where Final height and Initial height are the heights of treated plant at the day of harvesting and the height of the treated plant during the initial measurement, respectively.
2.3.3. Stress tolerance index
The stress tolerance index (TI ph ) was evaluated for plant height by comparing the mean height of treated plants to that of control plants under various heavy metal treatments for both species. This comparison was conducted using Eq. (3) [ 55 ]:
2.3.4. Fresh biomass and edible index
Fresh biomass from each plant part (leaves, stems and roots) was determined from both the harvested species. Additionally, the edible index was calculated to assess the edibility of the plants grown in various metal-spiked soil treatments. This calculation involved measuring the total fresh biomass of the species and the fresh biomass of the edible plant parts (leaves and stems), following the method outlined by Mi et al. [ 56 ], as per Eq. (4) :
where FW and FW e are the total fresh biomass of plant species and the edible fresh biomass of plants, such as leaves and stems, respectively. The calculation is based on the percentage ratio of FW e to FW.
2.3.5. Leaf area and root length
Leaf area (LA) and root length measurements were conducted to evaluate the potential impact of heavy metals on plant growth across various metal-spiked soil treatments. LA was determined by multiplying the maximum length (L) and width (W) of a leaf, as outlined in Eq. (5) [ 57 ]:
The root length was estimated by measuring the maximum length of the primary or main root, from the base to the root tip using a standard ruler.
2.4. Analytical procedures
The extraction procedure used for analyzing heavy metals in B. chinensis and B. rapa samples was based on the USEPA method 3050B [ 58 ]. Each plant sample was divided into leaves, stems and roots and analyzed separately to investigate the accumulation of spiked heavy metals. Plant samples were oven-dried at 105 °C for 2 h to eliminate moisture [ 39 ]. Subsequently, the dried samples were homogenized and ground using a pestle and mortar before passing through a 2 mm sieve.
Oven-dried plant samples (1 g) were digested with 15 mL HNO 3 (Sigma Aldrich, ACS reagent, 65 %), 10 mL H 2 O 2 (Sigma Aldrich, 30 %) and 10 mL HCl (Sigma Aldrich, ACS reagent, 35.4 %) for 2 h at 95 ± 5 °C. Following digestion, the samples were cooled down to ca . 24 °C and then diluted with distilled water. Subsequently, each sample was analyzed for Cd, Cr and Pb concentrations using an inductively coupled plasma-optical emission spectroscopy (ICP-OES Thermo Scientific iCAP 6000 Series, USA). Blank samples were prepared alongside each sample, and the instrument's accuracy was verified by analyzing the samples in triplicates. The operating conditions for ICP-OES used in this study are summarized as shown in Table 2 .
Operating conditions, parameters, limit of detection (LOD) and limit of quantification (LOQ) of Inductively Coupled Plasma-Optical Emission Spectroscopy (ICP-OES) used for analyzing heavy metal concentrations in Brassica species samples collected from pot experiments.
2.5. Statistical analysis
Statistical analyses for all heavy metals and plant parameters in B. chinensis and B. rapa were performed using a one-way analysis of variance (ANOVA) and TukeyHSD tests at 5 % significance level using SPSS (IBM SPSS Statistics 22) [ 59 ]. Moreover, the heavy metal concentrations in both Brassica species were used to derive the principal component analysis (PCA) of metal elements across the three different plant parts using SPSS.
3. Results and discussion
3.1. germination percentage.
The study examined the germination percentage to gauge the viability and resilience of seeds in response to adverse environmental conditions induced by heavy metals. This bioassay aimed to offer insights into the early stages of growth and establishment of B. chinensis and B. rapa seeds under different metal-spiked soils ( Fig. 4 ). In the control treatment, seeds of both species began to germinate around day 5 ± 1, while seeds in soils treated with metal exhibited a delay of at least 2 days (day 7 ± 1). This delay in germination time suggests the possibility of secondary induced dormancy in response to metal concentrations [ 60 ]. These current findings are consistent with those of Eze et al. [ 61 ], who similarly observed delayed germination in Cr-treated soils, with concentrations of up to 400 mg/kg compared to the control treatment, indicating that germination time increased with higher levels of metal contamination in soils.
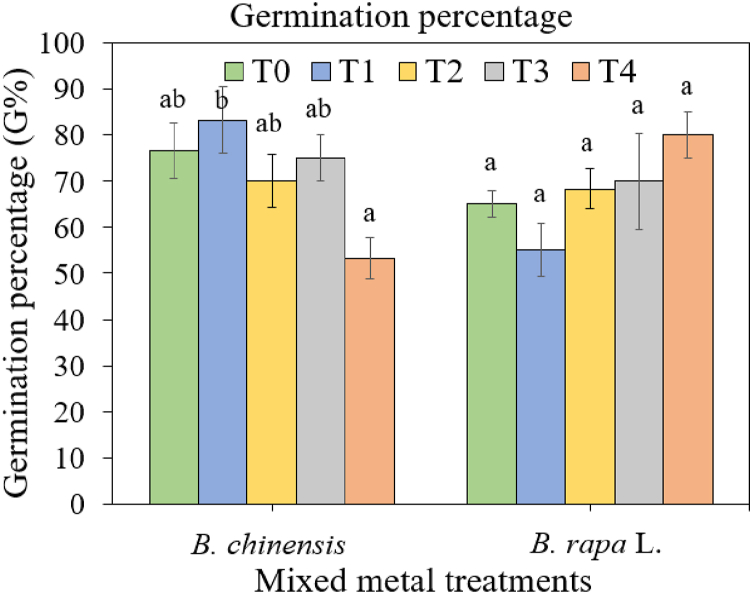
Germination percentage (%) of Brassica chinensis var. parachinensis and Brassica rapa L. under different soil metal treatments: T0: control soil, T1: 75 mg/kg Cd, Cr and Pb, T2: 150 mg/kg Cd, Cr and Pb, T3: 225 mg/kg Cd, Cr and Pb, and T4: 300 mg/kg Cd, Cr and Pb. Different letters represent significantly different means for each species after analyzing using one-way ANOVA and TukeyHSD tests at 5 % significance level.
The germination percentages of B. chinensis and B. rapa were significantly affected by the heavy metal treatments ( Fig. 4 ). The results indicated that B. chinensis maintained a consistent germination percentage ranging from 70 to 76 % across various treatments, except at 300 mg/kg (Treatment 4), where it significantly decreased to 53 %. Although the difference in germination percentage between the control and Treatment 4 was not significant, Treatment 4 exhibited a marked decrease compared to Treatment 2 (150 mg/kg Cd, Cr and Pb). Conversely, B. rapa displayed trend towards increased germination percentage, reaching levels up to 80 % at higher concentrations of Cd, Cr and Pb in the metal treatments, although these differences were not statistically significant.
The ANOVA results revealed contrasting germination percentages between the two Brassica species, highlighting their distinct sensitivity and tolerance to varying heavy metal concentrations in the soil [ 62 ]. This indicates that the germination of different plant seeds is influenced by different heavy metal concentrations [ 60 ]. The findings imply that elevated metal concentrations could impact the germination process of Brassica seeds. This study emphasizes the differential effects of heavy metal concentrations on the germination performance of Brassica seeds, consistent with findings from previous research [ 62 ].
3.2. Relative growth rate and stress tolerance index
The relative growth rate and stress tolerance index were assessed for both Brassica species to evaluate their growth performance under varying heavy metal treatments ( Fig. 5 ). The relative growth rate offers a quantitative assessment of the species' overall growth performance under different soil treatments, reflecting how heavy metals influence their development from seedling to maturity. Similarly, the stress tolerance index offers valuable information regarding the species’ ability to withstand adverse conditions caused by heavy metal toxicity. A decline in the stress tolerance index indicates a reduction in plant height relative to control conditions, highlighting the detrimental effects of heavy metals on growth and development. While both relative growth rate and stress tolerance index of B. chinensis ( Fig. 5 A and B) were significantly impacted by metal treatments, only the relative growth rate of B. rapa showed a significant effect ( Fig. 5 A). In the various spiked treatments, the relative growth rate of both species exhibited a decline in growth performance from 0 mg/kg (control) to increasing metal concentrations up to 300 mg/kg of Cd, Cr and Pb in the soil. Both B. chinensi s and B. rapa displayed a consistent decrease in growth rate from 0.05 cm/day to 0.04 cm/day with rising concentrations. However, the reduction in the relative growth rate for 300 mg/kg Cd, Cr and Pb (Treatment 4) was significantly different from the control treatment (T0) for both species.
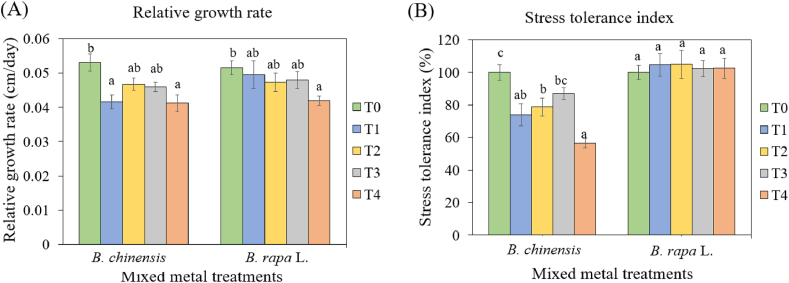
(A) Relative growth rate (RGR) and (B) stress tolerance index (TI ph ) of Brassica chinensis var. parachinensis and Brassica rapa L. under different soil metal treatments: T0: control soil, T1: 75 mg/kg Cd, Cr and Pb, T2: 150 mg/kg Cd, Cr and Pb, T3: 225 mg/kg Cd, Cr and Pb, and T4: 300 mg/kg Cd, Cr and Pb. Different letters represent significantly different means for each species following statistical analysis using one-way ANOVA and TukeyHSD tests at 5 % significance level.
However, the stress tolerance index exhibited contrasting patterns between B. chinensis and B. rapa ( Fig. 5 B). B. chinensis displayed a steep decline in the stress tolerance index, plummerting from 100 % to 56.6 % in response to spiked treatments with 300 mg/kg metals, while the stress tolerance index for B. rapa remained relatively steady across various metal treatments. Only the stress tolerance index of B. chinensis under all metal treatments (T1 to T4) was significantly lower than T0 (control treatment), whereas this difference was not observed for B. rapa . This indicates that the relative growth rate of B. chinensis was significantly hindered by the presence of heavy metals, suggesting that metal stress was specific to this Brassica species, not B. rapa . B. rapa accumulated the highest concentrations of Cd and Pb, supporting the notion that it demonstrates high tolerance to elevated soil concentrations of Cd, Pb and Cr [ 38 ]. In B. chinensis , the transport of heavy metals from the roots to the shoots could disrupt cellular metabolism, contributing to the decline in growth rate and subsequent slowdown in growth performance [ 60 ]. Elevated concentrations of heavy metals in the soil have been shown to distress shoot development, affecting plant height, and potentially influencing root growth, thereby reducing nutrient and water uptake by the plants [ 63 , 64 ].
3.3. Fresh biomass and edible index
Fresh biomass of B. chinensis and B. rapa provided quantitative data on the total plant biomass, including the leaves, stems and roots ( Fig. 6 A and C), reflecting the overall growth and development of the species under different soil treatments. On the other hand, the edible index determined the proportion of edible biomass (leaves and stems) affected by varying levels of heavy metal contaminations ( Fig. 6 B and D). B. chinensis consistently exhibited higher total fresh biomass (18.2–22.6 g/plant) across all control and treated soils compared to B. rapa (15.2–18.6 g/plant). Furthermore, B. chinensis yielded greater root biomass (1.06–1.33 g/plant) across the various spiked treatments compared to B. rapa (0.38–0.58 g/plant), leading to a higher edible index of over 97.0 % in B. rapa compared to the edible index in B. chinensis (92.9–94.8 %). However, this study observed insignificant differences in the fresh biomass of leaf, stem and root parts, as well as edible indices of both species under various soil treatments. The observed decline in relative growth rate in both species ( Fig. 5 A) and stress tolerance index in B. chinensis did not correlate with a decrease in fresh biomass and edible index. It is possible that dry biomass could have been influenced by metal concentrations, as reported by studies by Amin et al. (2013). Unfortunately, this study did not evaluate dry biomass. Overall, these findings suggest that the two Brassica species, particularly B. rapa , might demonstrate tolerance to Cd, Cr and Pb heavy metals ( Fig. 4 , Fig. 5 , Fig. 6 ), implying their potential ability to thrive in toxic soil environments [ 60 ].
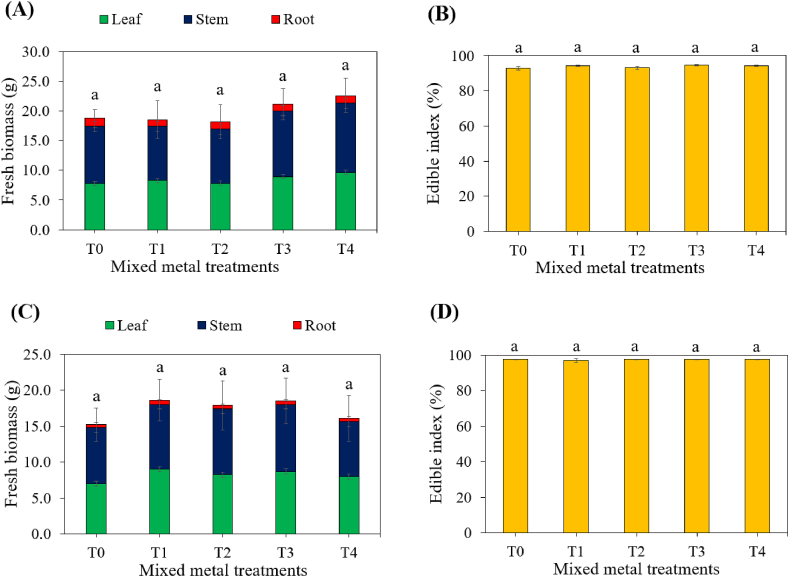
Fresh biomass and edible indices of (A–B) B. chinensis var. parachinensis , respectively, and (C–D) B. rapa , L., respectively, under different spiked soil treatments: T0: control soil, T1: 75 mg/kg Cd, Cr and Pb, T2: 150 mg/kg Cd, Cr and Pb, T3: 225 mg/kg Cd, Cr and Pb, and T4: 300 mg/kg Cd, Cr and Pb. Different letters represent significantly different means for each species after analyzing using one-way ANOVA and TukeyHSD tests at 5 % significance level.
3.4. Leaf area and root length
The average leaf areas and root lengths of B. chinensis and B. rapa were measured to investigate the effects of heavy metals on the physiological growth of Brassica plants and the adaptation of species to grow effectively in contaminated environments ( Fig. 7 A and B). Leaf areas for B. chinensis and B. rapa showed an increase of 23.5 % and 11.8 %, respectively, in the highest soil treatment compared to the control soils, although these differences were not statistically significant ( Fig. 7 A). While there were no significant differences in leaf areas of B. chinensis , a trend toward larger sizes was observed with increasing soil-metal treatments, up to 300 mg/kg metals. Conversely, consistent leaf areas were observed for B. rapa across different soil treatments. No visible signs of toxicity were detected on the leaves of either species, suggesting potential tolerance or adaptive mechanisms to high metal concentrations, particularly in B. rapa [ 65 ].
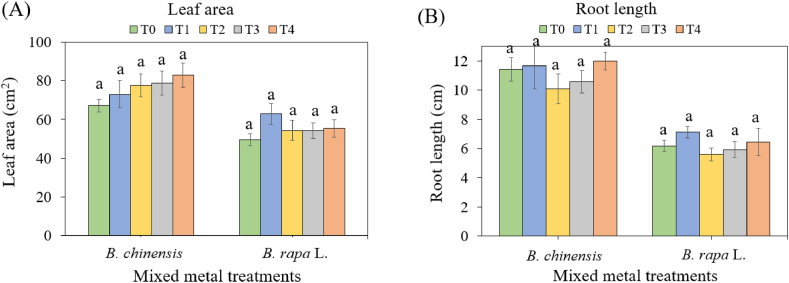
(A) Leaf areas and (B) root lengths of Brassica chinensis var. parachinensis and Brassica rapa L. under different soil metal treatments: T0: control soil, T1: 75 mg/kg Cd, Cr and Pb, T2: 150 mg/kg Cd, Cr and Pb, T3: 225 mg/kg Cd, Cr and Pb, and T4: 300 mg/kg Cd, Cr and Pb. Different letters represent significantly different means for each species following analysis using one-way ANOVA and TukeyHSD tests at 5 % significance level.
The average maximum root lengths for B. chinensis and B. rapa across all soil treatments were 11.2 cm and 6.26 cm, respectively ( Fig. 7 B). However, neither species exhibited symptoms of metal contamination, and there were no significant differences in root lengths from the control soil (T0) to the highest soil treatment (T4). Additionally, there were no notable differences between root lengths for both species across different soil treatments, indicating their ability to grow and absorb nutrients normally under stress conditions. Despite a significant reduction in the relative growth rate for both species, this did not translate in significant differences in leaf areas and root lengths, mirroring the findings on fresh biomass and edible index. In theory, a decline in the relative growth rate should negatively impact fresh biomass, edible index, leaf area and root length [ 51 ]. Leaf area development and plant growth are typically influenced by the division and enlargement of plant cells, processes that could adversely affected by the presence of Cd and Pb [ 66 ]. However, the stimulatory effect of Cd in leaves could be attributed to the plants’ defense mechanism, where they synthesize stress proteins and secondary metabolites, alter antioxidant enzyme activity and reduce oxidative stress [ [67] , [68] , [69] ]. These results suggest that both B. chinensis (to a certain extent, given its low stress tolerance index) and B. rapa may exhibit tolerance to metal stress conditions, making them potential candidates for soil remediation techniques.
3.5. Heavy metals in plant species
Table 3 presents the concentrations of heavy metals in various plant parts (leaf, stem and root) of B. chinensis and B. rapa across different soil treatments (0 mg/kg – 300 mg/kg Cd, Cr and Pb). Heavy metal contents in different parts of Brassica plants were measured to understand how these plants absorb and accumulate toxins from the soil, assessing their ability to tolerate and potentially remediate contaminated environments. However, the duration of the pot experiment was only 60 days, which is relatively short to fully capture the long-term effects of heavy metal exposure on plant growth and development. Among the soil treatments (T1 – T4), B. rapa exhibited the highest accumulation of Cd, with 864 mg/kg Cd in root of T4, compared to B. chinensis , which accumulated 739 mg/kg Cd in root of T4. Notably, Cd tended to accumulate significantly in the roots of both species across all treatments, except in the control soils where no significant differences were observed. Previous studies have shown that Cd tends to distribute evenly across all plant parts in various species under natural conditions [ 38 ]. The sequestration of Cd in root vacuoles, particularly when exposed to high soil Cd levels, suggests the presence of Cd transporters as detoxification mechanisms in these Brassica species. These mechanisms facilitate the uptake and localization of Cd, minimizing its exposure in shoots [ [70] , [71] , [72] ]. Under toxic conditions, root vacuoles play a crucial role in storing ions and metabolites, contributing to the detoxification process and normal cell development [ 73 ]. These findings underscore the potential and tolerance of Brassica species, particularly B. rapa , to germinate and thrive in toxic environments.
Mean heavy metal concentrations (standard deviation, SD) in the different plant parts (leaf, stem and root) of Brassica chinensis var. parachinensis and Brassica rapa L. Different letters per heavy metal (row) represent significantly different means for the different parts (leaf, stem, and root) for each species following analysis using one-way ANOVA and TukeyHSD tests at 5 % significance level.
The patterns of Cr contents in both B. chinensis and B. rapa reflected the accumulations of Cd in different plant parts (leaf, stem and root) across the various soil metal treatments, with Cr significantly accumulating in the root part of both species. There were no significant differences observed between the leaf and stem parts in both the Brassica species, indicating minimal Cr uptake in the aerial parts compared to the roots. Both species accumulated similar amounts of Cr in different plant parts, with B. chinensis and B. rapa accumulating the highest amounts in the root parts at 161 mg/kg and 132 mg/kg, respectively. Cr, being a non-essential heavy metal, did not have any known biological function in plant physiology, leading to the absence of a specific uptake mechanism for Cr in plants [ 74 , 75 ]. The translocation and distribution of Cr are influenced by factors such as plant species, Cr concentration in the growth medium and its oxidation state and due to its low mobility in plant roots, the concentration of Cr is 100 times higher in roots than in aerial parts of plants [ 62 , 76 , 77 ]. In this study, Cr concentration was observed to be the highest in the roots, which could be attributed to the sequestration of Cr in the vacuoles of root cells as a protective mechanism [ 78 ], thereby naturally enhancing the tolerance of the two species to Cr toxicity [ 79 ].
Significant differences were observed in the various plant parts (leaf, stem and root) of B. chinensis and B. rapa ( Table 3 ), with notable Pb accumulation detected in both the leaf and root parts of both species. This suggests that both species possess the capability to accumulate substantial amounts of Pb in their aerial parts. At spiked Pb concentrations of 300 mg/kg in soil (T4), both B. chinensis and B. rapa accumulated up to 406 mg/kg and 340 mg/kg Pb, respectively ( Table 3 ), in the leaf part of the plants, indicating the high mobility of Pb in Brassica species. B. chinensis exhibited the highest Pb content (953 mg/kg Pb) in the root part of the plant compared to B. rapa . Some Brassica species may regulate the uptake of highly mobile Pb through intracellular detoxification involving sequestration and cell binding in plant cells to reduce the translocation of Pb to the aerial parts of the plants [ 62 , [80] , [81] , [82] ]. Although Pb tends to highly mobile at lower soil pH and is typically found in water-soluble complexes such as CdSO 4 compounds, CdCl + , CdHCO 3 + cations and Pb 2+ [ 83 , 84 ], this study demonstrated that Pb could readily accumulate in the plant parts of Brassica . These findings suggest that both Brassica species have the potential to accumulate significant amounts of Cd, Cr and Pb and could be cultivated in contaminated soil environments containing up to 300 mg/kg of heavy metals. Future studies could also assess the potential impacts of heavy metal contamination on soil microbial communities, which could play a crucial role in nutrient cycling and plant health, to further understand the limitations of these species.
4. Conclusion
Previous studies have highlighted the phytoremediation potential of these species, but their specific responses to heavy metal treatments based on their morphological, physiological and biochemical traits have been relatively understudied. The findings indicate that B. rapa and, to some extent, B. chinensis possess the capacity to accumulate significant amounts of non-essential heavy metals (Cd, Cr and Pb) from soils, reflecting their tolerance to toxic soil conditions, which is crucial for soil remediation efforts. Germination percentages for both the Brassica species were consistent across various soil treatments, albeit with delays in more toxic environments. The relative growth rate notably decreased at higher soil treatments, highlighting the impact of heavy metals on Brassica growth rate, particularly evident at 300 mg/kg Cd, Cr and Pb concentrations. The stress tolerance index suggested a substantial decline in B. chinensis’ plant heights compared to controls, whereas B. rapa exhibited stability, suggesting its resilience to toxic conditions. Despite insignificant differences due to varied metal treatments, B. chinensis exhibited higher fresh biomass compared to B. rapa , with B. chinensis also displaying greater root biomass. Leaf areas increased with higher soil treatments for both species, while root lengths showed no significant differences, indicating their tolerance to high metal concentrations. B. rapa accumulated the highest amount of Cd (864 mg/kg Cd), while B. chinensis accumulated the most Pb (953 mg/kg Pb), primarily in the root parts. Both species similarly accumulated Cr in the roots. The hypothesis underlying this research posited that B. chinensis and B. rapa would exhibit varying degrees of tolerance and accumulation of Cd, Cr, and Pb in response to increasing concentrations of selected heavy metals in soil. Further investigations through long-term effects field studies can provide insights into the effects of heavy metal contamination on both soil and plant health in real-world agricultural settings. The study suggests that both Brassica species, particularly B. rapa , exhibit high tolerance to non-essential heavy metals and can thrive in toxic soil conditions, potentially serving as hyperaccumulators for green remediation techniques.

Data availability statement
Data will be made available on request.
CRediT authorship contribution statement
Adzrin Asikin Zunaidi: Writing – review & editing, Writing – original draft, Visualization, Validation, Project administration, Methodology, Investigation, Funding acquisition, Formal analysis, Data curation, Conceptualization. Lee Hoon Lim: Writing – review & editing, Visualization, Supervision, Resources, Project administration, Funding acquisition, Data curation, Conceptualization. Faizah Metali: Writing – review & editing, Visualization, Supervision, Resources, Project administration, Funding acquisition, Data curation, Conceptualization.
Declaration of competing interest
The authors declare that they have no known competing financial interests or personal relationships that could have appeared to influence the work reported in this paper.
- 1. Sanjosé I., Navarro-Roldán F., Infante-Izquierdo M.D., Martínez-Sagarra G., Devesa J.A., Polo A., Ramírez-Acosta S., Sánchez-Gullón E., Jiménez-Nieva F.J., Muñoz-Rodríguez A.F. Accumulation and effect of heavy metals on the germination and growth of salsola vermiculata l. seedlings, Diversity. 2021;13:1–15. doi: 10.3390/d13110539. [ DOI ] [ Google Scholar ]
- 2. Shen X., Dai M., Yang J., Sun L., Tan X., Peng C., Ali I., Naz I. A critical review on the phytoremediation of heavy metals from environment: performance and challenges. Chemosphere. 2022;291 doi: 10.1016/j.chemosphere.2021.132979. [ DOI ] [ PubMed ] [ Google Scholar ]
- 3. Hassaan M.A., El Nemr A., Madkour F.F. Environmental assessment of heavy metal pollution and human health risk. Am. J. Water Sci. Eng. 2016;2:14–19. doi: 10.11648/j.ajwse.20160203.11. [ DOI ] [ Google Scholar ]
- 4. Seneviratne M., Rajakaruna N., Rizwan M., Madawala H.M.S.P., Ok Y.S., Vithanage M. Heavy metal-induced oxidative stress on seed germination and seedling development: a critical review. Environ. Geochem. Health. 2019;41:1813–1831. doi: 10.1007/s10653-017-0005-8. [ DOI ] [ PubMed ] [ Google Scholar ]
- 5. Wu G., Kang H., Zhang X., Shao H., Chu L., Ruan C. A critical review on the bio-removal of hazardous heavy metals from contaminated soils: Issues, progress, eco-environmental concerns and opportunities, J. Hazard. Mater. 2010;174:1–8. doi: 10.1016/j.jhazmat.2009.09.113. [ DOI ] [ PubMed ] [ Google Scholar ]
- 6. Suman J., Uhlik O., Viktorova J., Macek T. Phytoextraction of heavy metals: a promising tool for clean-up of polluted environment? Front. Plant Sci. 2018;871 doi: 10.3389/fpls.2018.01476. [ DOI ] [ PMC free article ] [ PubMed ] [ Google Scholar ]
- 7. Yan A., Wang Y., Tan S.N., Mohd Yusof M.L., Ghosh S., Chen Z. Phytoremediation: a promising approach for revegetation of heavy metal-polluted land. Front. Plant Sci. 2020;11:1–15. doi: 10.3389/fpls.2020.00359. [ DOI ] [ PMC free article ] [ PubMed ] [ Google Scholar ]
- 8. Rigoletto M., Calza P., Gaggero E., Malandrino M., Fabbri D. Bioremediation methods for the recovery of lead-contaminated soils: a review. Appl. Sci. 2020;10 doi: 10.3390/app10103528. [ DOI ] [ Google Scholar ]
- 9. Nouri H., Chavoshi Borujeni S., Nirola R., Hassanli A., Beecham S., Alaghmand S., Saint C., Mulcahy D. Application of green remediation on soil salinity treatment: a review on halophytoremediation. Process Saf. Environ. Protect. 2017;107:94–107. doi: 10.1016/j.psep.2017.01.021. [ DOI ] [ Google Scholar ]
- 10. Jayasri M.A., Suthindhiran K. Effect of zinc and lead on the physiological and biochemical properties of aquatic plant Lemna minor: its potential role in phytoremediation. Appl. Water Sci. 2017;7:1247–1253. doi: 10.1007/s13201-015-0376-x. [ DOI ] [ Google Scholar ]
- 11. Bae J., Benoit D.L., Watson A.K. Effect of heavy metals on seed germination and seedling growth of common ragweed and roadside ground cover legumes. Environ. Pollut. 2016;213:112–118. doi: 10.1016/j.envpol.2015.11.041. [ DOI ] [ PubMed ] [ Google Scholar ]
- 12. Lefèvre I., Marchal G., Corréal E., Zanuzzi A., Lutts S. Variation in response to heavy metals during vegetative growth in Dorycnium pentaphyllum Scop. Plant Growth Regul. 2009;59:1–11. doi: 10.1007/s10725-009-9382-z. [ DOI ] [ Google Scholar ]
- 13. Moosavi S.A., Gharineh M.H., Tavakkol Afshari R., Ebrahimi A. Effects of some heavy metals on seed germination characteristics of canola (Barassica napus), wheat (Triticum aestivum) and safflower (Carthamus tinctorious) to evaluate phytoremediation potential of these crops. J. Agric. Sci. 2012;4:11–19. doi: 10.5539/jas.v4n9p11. [ DOI ] [ Google Scholar ]
- 14. Márquez-García B., Márquez C., Sanjosé I., Nieva F.J.J., Rodríguez-Rubio P., Muñoz-Rodríguez A.F. The effects of heavy metals on germination and seedling characteristics in two halophyte species in Mediterranean marshes. Mar. Pollut. Bull. 2013;70:119–124. doi: 10.1016/j.marpolbul.2013.02.019. [ DOI ] [ PubMed ] [ Google Scholar ]
- 15. Peralta J.R., Gardea-Torresdey J.L., Tiemann K.J., Gomez E., Arteaga S., Rascon E., Parsons J.G. Uptake and effects of five heavy metals on seed germination and plant growth in Alfalfa (L.) Bull. Environ. Contam. Toxicol. 2001;66:727–734. doi: 10.1007/s00128-001-0069-z. [ DOI ] [ PubMed ] [ Google Scholar ]
- 16. Singh D., Nath K., Sharma Y.K. Response of wheat seed germination and seedling growth under copper stress. J. Environ. Biol. 2007;28:409–414. [ PubMed ] [ Google Scholar ]
- 17. Aas R.Ø. Availability of plant nutrients under conventional farming and conservation farming with and without biochar : a field study of maize on acidic. sandy loam in Zambia. 2016;52 https://brage.bibsys.no/xmlui/handle/11250/2435230 [ Google Scholar ]
- 18. Anjum N.A., Ahmad I., Válega M., Mohmood I., Gill S.S., Tuteja N., Duarte A.C., Pereira E. Salt marsh halophyte services to metal-metalloid remediation: assessment of the processes and underlying mechanisms. Crit. Rev. Environ. Sci. Technol. 2014;44:2038–2106. doi: 10.1080/10643389.2013.828271. [ DOI ] [ Google Scholar ]
- 19. Castro R., Pereira S., Lima A., Corticeiro S., Válega M., Pereira E., Duarte A., Figueira E. Accumulation, distribution and cellular partitioning of mercury in several halophytes of a contaminated salt marsh. Chemosphere. 2009;76:1348–1355. doi: 10.1016/j.chemosphere.2009.06.033. [ DOI ] [ PubMed ] [ Google Scholar ]
- 20. Reboreda R., Caçador I. Halophyte vegetation influences in salt marsh retention capacity for heavy metals. Environ. Pollut. 2007;146:147–154. doi: 10.1016/j.envpol.2006.05.035. [ DOI ] [ PubMed ] [ Google Scholar ]
- 21. Duarte B., Caetano M., Almeida P.R., Vale C., Caçador I. Accumulation and biological cycling of heavy metal in four salt marsh species, from Tagus estuary (Portugal) Environ. Pollut. 2010;158:1661–1668. doi: 10.1016/j.envpol.2009.12.004. [ DOI ] [ PubMed ] [ Google Scholar ]
- 22. Válega M., Lillebø A.I., Pereira M.E., Caçador I., Duarte A.C., Pardal M.A. Mercury in salt marshes ecosystems: Halimione portulacoides as biomonitor. Chemosphere. 2008;73:1224–1229. doi: 10.1016/j.chemosphere.2008.07.053. [ DOI ] [ PubMed ] [ Google Scholar ]
- 23. Mahar A., Wang P., Ali A., Awasthi M.K., Lahori A.H., Wang Q., Li R., Zhang Z. Challenges and opportunities in the phytoremediation of heavy metals contaminated soils: a review. Ecotoxicol. Environ. Saf. 2016;126:111–121. doi: 10.1016/j.ecoenv.2015.12.023. [ DOI ] [ PubMed ] [ Google Scholar ]
- 24. Alkorta I., Hernández-Allica J., Becceril J.M., Amezaga I., Albizu I., Onaindia M., Garbisu C. Chelate-enhanced phytoremediation of soils polluted with heavy metals. Rev. Environ. Sci. Biotechnol. 2004;3:55–70. doi: 10.1016/j.envpol.2007.11.015. [ DOI ] [ Google Scholar ]
- 25. Bhargava A., Carmona F.F., Bhargava M., Srivastava S. Approaches for enhanced phytoextraction of heavy metals. J. Environ. Manag. 2012;105:103–120. doi: 10.1016/j.jenvman.2012.04.002. [ DOI ] [ PubMed ] [ Google Scholar ]
- 26. Leštan D., Luo C., Li X. The use of chelating agents in the remediation of metal-contaminated soils: a review. Environ. Pollut. 2008;153:3–13. doi: 10.1016/j.envpol.2007.11.015. [ DOI ] [ PubMed ] [ Google Scholar ]
- 27. Padmavathiamma P.K., Li L.Y. Phytoremediation technology: Hyper-accumulation metals in plants. Water Air Soil Pollut. 2007;184:105–126. doi: 10.1007/s11270-007-9401-5. [ DOI ] [ Google Scholar ]
- 28. Kacálková L., Tlustoš P., Száková J. Phytoextraction of risk elements by willow and poplar trees. Int. J. Phytoremediation. 2015;17:414–421. doi: 10.1080/15226514.2014.910171. [ DOI ] [ PubMed ] [ Google Scholar ]
- 29. Pulford I.D., Watson C. Phytoremediation of heavy metal-contaminated land by trees - a review. Environ. Int. 2003;29:529–540. doi: 10.1016/S0160-4120(02)00152-6. [ DOI ] [ PubMed ] [ Google Scholar ]
- 30. Tlustoš P., Pavlíková D., Száková J., Fischeroá Z., Balík J. Exploitation of fast growing trees in metal remediation. Phytoremediation Rhizoremediation. 2006:83–102. doi: 10.1007/978-1-4020-4999-4_7. [ DOI ] [ Google Scholar ]
- 31. Brunner I., Luster J., Günthardt-Goerg M.S., Frey B. Heavy metal accumulation and phytostabilisation potential of tree fine roots in a contaminated soil. Environ. Pollut. 2008;152:559–568. doi: 10.1016/j.envpol.2007.07.006. [ DOI ] [ PubMed ] [ Google Scholar ]
- 32. Rajakaruna N., Gall J.E. In: Brassicaceae. Lang M., editor. Nova Science Publishers, Inc.; ME, US: 2016. The physiology, functional genomics, and applied ecology of heavy metal-tolerant Brassicaceae. [ Google Scholar ]
- 33. Neilson S., Rajakaruna N. In: Plant Fam. Brassicaceae Contrib. Towar. Phytoremediation, Environ. Pollut. Anjum N.A., et al., editors. Springer Science+Business Media B.V., ME, US; 2012. Roles of rhizospheric processes and plant physiology in applied phytoremediation of contaminated soils using Brassica oilseeds; pp. 313–330. [ DOI ] [ Google Scholar ]
- 34. Hernández-Allica J., Becerril J.M., Garbisu C. Assessment of the phytoextraction potential of high biomass crop plants. Environ. Pollut. 2008;152:32–40. doi: 10.1016/j.envpol.2007.06.002. [ DOI ] [ PubMed ] [ Google Scholar ]
- 35. Mourato M.P., Moreira I.N., Leitão I., Pinto F.R., Sales J.R., Martins L.L. Effect of heavy metals in plants of the genus Brassica. Int. J. Mol. Sci. 2015;16:17975–17998. doi: 10.3390/ijms160817975. [ DOI ] [ PMC free article ] [ PubMed ] [ Google Scholar ]
- 36. Purakayastha T.J., Viswanath T., Bhadraray S., Chhonkar P.K., Adhikari P.P., Suribabu K. Phytoextraction of zinc, copper, nickel and lead from a contaminated soil by different species of Brassica. Int. J. Phytoremediation. 2008;10:61–72. doi: 10.1080/15226510701827077. [ DOI ] [ PubMed ] [ Google Scholar ]
- 37. Zunaidi A.A., Lim L.H., Metali F. Transfer of heavy metals from soils to curly mustard (Brassica juncea (L.) Czern.) grown in an agricultural farm in Brunei Darussalam. Heliyon. 2021;7 doi: 10.1016/j.heliyon.2021.e07945. [ DOI ] [ PMC free article ] [ PubMed ] [ Google Scholar ]
- 38. Zunaidi A.A., Lim L.H., Metali F. Comparative assessment of the heavy metal phytoextraction potential of vegetables from agricultural soils : a field experiment. Heliyon. 2023;9 doi: 10.1016/j.heliyon.2023.e13547. [ DOI ] [ PMC free article ] [ PubMed ] [ Google Scholar ]
- 39. Zunaidi A.A., Lim L.H., Metali F. Assessments of heavy metals in commercially available fertilizers in Brunei Darussalam. Agric. Res. 2021;10:234–242. doi: 10.1007/s40003-020-00500-4. [ DOI ] [ Google Scholar ]
- 40. Mongkhonsin B., Nakbanpote W., Meesungnoen O., Prasad M.N.V. In: Cadmium Toxic. Toler. Plants from Physiol. To Remediat. Hasanuzzaman M., Prasad M.N.V., Fujita M., editors. Elsevier Inc.; 2019. Adaptive and tolerance mechanisms in herbaceous plants exposed to cadmium; pp. 73–109. [ DOI ] [ Google Scholar ]
- 41. Williams P.H., Hill C.B. Rapid-cycling populations of Brassica. Science. 1986;13(80):1385–1389. doi: 10.1126/science.232.4756.1385. [ DOI ] [ PubMed ] [ Google Scholar ]
- 42. Storer D.A. Terrific Science Press; Ohio, USA: 2005. The Chemistry of Soil Analysis. [ Google Scholar ]
- 43. Allen S.E., Grimshaw H.M., Parkinson J.A., Quarmby C. Blackwell Scientific Publications; UK: Oxford: 1989. Chemical Analysis of Ecological Materials. [ Google Scholar ]
- 44. Hanlon E.A. Soil pH and electrical conductivity: a county extension soil laboratory manual. Cirl1081. UF/IFAS Ext. 2015:1–10. http://edis.ifas.ufl.edu/ss118 [ Google Scholar ]
- 45. Van Reeuwijk L. International Soil Reference and Information Centre. sixth ed. Food and Agriculture Organization of the United Nations; 2002. Procedures for soil analysis. [ Google Scholar ]
- 46. Bojko O., Kabala C. Loss-on-ignition as an estimate of total organic carbon in the mountain soils. J. Soil Sci. 2014;47:71–79. [ Google Scholar ]
- 47. Amacher M.C., Neill K.P.O., Dresbach R., Palmer C. 2003rd ed. 2003. Forest Inventory & Analysis: Manual of Soil Analysis Methods. [ Google Scholar ]
- 48. GumbaraDarmawan R.H., Sumawinata B. A comparison of cation exchange capacity of organic soils determined by ammonium acetate solutions buffered at some pHs ranging between around field pH and 7.0. Earth Environ. Sci. 2019 doi: 10.1088/1755-1315/393/1/012015. [ DOI ] [ Google Scholar ]
- 49. IUSS Working Group WRB . fourth ed. International Union of Soil Sciences (IUSS); Vienna, Austria: 2022. World Reference Base for Soil Resources. International Soil Classification System for Naming Soils and Creating Legends for Soil Maps; p. 234. [ Google Scholar ]
- 50. Mkumbo S. Development of a low cost remediation method for heavy metal polluted soils. TRITA LWR Licentiate Thesis 2067, Stockholm. 2012 [ Google Scholar ]
- 51. Amin H., Arain B.A., Amin F., Surhio M.A. Phytotoxicity of chromium on germination, growth and biochemical attributes of Hibiscus esculentus L. Am. J. Plant Sci. 2013;4:2431–2439. doi: 10.4236/ajps.2013.412302. [ DOI ] [ Google Scholar ]
- 52. Feller C., Bleiholder H., Frau E., Hess M., Wicke H., Meier U., van den Boom T., Stauss R., Klose F.R., Hack H., Buhr F.L., Lancashire P.D. 2001st ed. BBCH monograph; 1994. Growth Stages of Mono-And Dicotyledonous Plants. [ Google Scholar ]
- 53. Bahira S., Behera S., Pattnaik G., Puhan P. Phytoremediation of chromium by chickpea (Ciecer arietinum L.) and mung bean (Vigna radiata L.) A comparative study. Int. J. Curr. Sci. Res. 2018;4:1589–1598. [ Google Scholar ]
- 54. Hunt R., Causton D.R., Shipley B., Askew A.P. A modern tool for classical plant growth analysis. Ann. Bot. 2002;90:485–488. doi: 10.1093/aob/mcf214. [ DOI ] [ PMC free article ] [ PubMed ] [ Google Scholar ]
- 55. Wilkins D.A. The measurement of tolerance to edaphic factors by means of root growth. New Phytol. 1978;80:623–633. doi: 10.1111/j.1469-8137.1978.tb01595.x. [ DOI ] [ Google Scholar ]
- 56. Mi B., Liu F., Xie L., Zhou H., Wu F., Dai X. Evaluation of the uptake capacities of heavy metals in Chinese cabbage. Ecotoxicol. Environ. Saf. 2019;171:511–517. doi: 10.1016/j.ecoenv.2019.01.022. [ DOI ] [ PubMed ] [ Google Scholar ]
- 57. Chanda S.V., Singh Y.D. Estimation of leaf area in wheat using linear measurements, Plant Breed. Seed Sci. 2002;46 [ Google Scholar ]
- 58. USEPA . 1996. EPA Method 3050B (SW-846): Acid Digestion of Sediments, Sludges, and Soils, 1996; pp. 1–12. [ DOI ] [ Google Scholar ]
- 59. SPSS, IBM Corp . IBM Corp.; New York: 2018. IBM SPSS Statistics for Windows, Version 22 (2018) Amonk, NY. [ Google Scholar ]
- 60. Ongon’g R.O., Edokpayi J.N., Msagati T.A.M., Tavengwa N.T., Ijoma G.N., Odiyo J.O. The potential health risk associated with edible vegetables grown on cr(VI) polluted soils. Int. J. Environ. Res. Publ. Health. 2020;17 doi: 10.3390/ijerph17020470. [ DOI ] [ PMC free article ] [ PubMed ] [ Google Scholar ]
- 61. Eze C.N., Odoh C.K., Eze E.A., Orjiakor P.I., Enemuor S.C., Okobo U.J. Chromium (III) and its effects on soil microbial activities and phytoremediation potentials of Arachis hypogea and Vigna unguiculata. Afr. J. Biotechnol. 2018;17:1207–1214. doi: 10.5897/ajb2018.16566. [ DOI ] [ Google Scholar ]
- 62. Shahid M., Shamshad S., Rafiq M., Khalid S., Bibi I., Niazi N.K., Dumat C., Rashid M.I. Chromium speciation, bioavailability, uptake, toxicity and detoxification in soil-plant system: a review. Chemosphere. 2017;178:513–533. doi: 10.1016/j.chemosphere.2017.03.074. [ DOI ] [ PubMed ] [ Google Scholar ]
- 63. Bahira S., Behera S., Puhan P. Phytoremediation of chromium by chickpea (Ciecer arietinum L .) and it's toxic effect. Remarking an Anal. 2018;3:80–85. [ Google Scholar ]
- 64. Sundarmoorthy P., Sankarganesh K., Selvaraj M., Baskaran L. Chromium induced changes in Soybean (Glycine max L.) metabolism. World Sci. News. 2015;16:145–178. [ Google Scholar ]
- 65. Piršelová B., Kubová V., Boleček P., Hegedusová A. Impact of cadmium toxicity on leaf area and stomatal characteristics in Faba Bean. J. Microbiol. Biotechnol. Food Sci. 2021;11:1–4. doi: 10.15414/jmbfs.3718. [ DOI ] [ Google Scholar ]
- 66. Hatamian M., Rezaei Nejad A., Kafi M., Souri M.K., Shahbazi K. Interaction of lead and cadmium on growth and leaf morphophysiological characteristics of European hackberry (Celtis australis) seedlings. Chem. Biol. Technol. Agric. 2020;7:1–8. doi: 10.1186/s40538-019-0173-0. [ DOI ] [ Google Scholar ]
- 67. Lai H.Y. Effects of leaf area and transpiration rate on accumulation and compartmentalization of cadmium in Impatiens walleriana. Water Air Soil Pollut. 2015;226 doi: 10.1007/s11270-014-2246-9. [ DOI ] [ Google Scholar ]
- 68. Allender W.J., Cresswell G.C., Kaldor J., Kennedy I.R. Effect of lithium and lanthanum on herbicide induced hormesis in hydroponically-grown cotton and corn. J. Plant Nutr. 1997;20:81–95. doi: 10.1080/01904169709365235. [ DOI ] [ Google Scholar ]
- 69. Calabrese E.J., Blain R.B. Hormesis and plant biology. Environ. Pollut. 2009;157:42–48. doi: 10.1016/j.envpol.2008.07.028. [ DOI ] [ PubMed ] [ Google Scholar ]
- 70. Huang X., Duan S., Wu Q., Yu M., Shabala S. Reducing cadmium accumulation in plants: structure – Function relations and tissue-specific operation of transporters in the spotlight. Plants. 2020;9:18. doi: 10.3390/plants9020223. [ DOI ] [ PMC free article ] [ PubMed ] [ Google Scholar ]
- 71. Ueno D., Yamaji N., Kono I., Huang C.F., Ando T., Yano M., Ma J.F. Gene limiting cadmium accumulation in rice. Proc. Natl. Acad. Sci. U.S.A. 2010;107:16500–16505. doi: 10.1073/pnas.1005396107. [ DOI ] [ PMC free article ] [ PubMed ] [ Google Scholar ]
- 72. Kopittke P.M., de Jonge M.D., Wang P., Mckenna B.A., Lombi E., Paterson D.J., Howard D.L., James S.A., Spiers K.M., Ryan C.G., Johnson A.A.T., Menzies N.W. Laterally resolved speciation of arsenic in roots of wheat and rice using fluorescence-XANES imaging. New Phytol. 2014;201:1251–1262. doi: 10.1111/nph.12595. [ DOI ] [ PubMed ] [ Google Scholar ]
- 73. Martinoia E., Meyer S., De Angeli A., Nagy R. Vacuolar transporters in their physiological context. Annu. Rev. Plant Biol. 2012;63:183–213. doi: 10.1146/annurev-arplant-042811-105608. [ DOI ] [ PubMed ] [ Google Scholar ]
- 74. Oliveira H. Chromium as an environmental pollutant: insights on induced plant toxicity. J. Bot., Le. 2012;2012:1–8. doi: 10.1155/2012/375843. [ DOI ] [ Google Scholar ]
- 75. Sharma A., Kapoor D., Wang J., Shahzad B., Kumar V., Bali A.S., Jasrotia S., Zheng B., Yuan H., Yan D. Chromium bioaccumulation and its impacts on plants: an overview. Plants. 2020;9:1–17. doi: 10.3390/plants9010100. [ DOI ] [ PMC free article ] [ PubMed ] [ Google Scholar ]
- 76. Shanker A.K., Cervantes C., Loza-Tavera H., Avudainayagam S. Chromium toxicity in plants. Environ. Int. 2005;31:739–753. doi: 10.1016/j.envint.2005.02.003. [ DOI ] [ PubMed ] [ Google Scholar ]
- 77. Gupta A.K., Sinha S. Chemical fractionation and heavy metal accumulation in the plant of Sesamum indicum (L.) var. T55 grown on soil amended with tannery sludge: selection of single extractants. Chemosphere. 2006;64:161–173. doi: 10.1016/j.chemosphere.2005.10.016. [ DOI ] [ PubMed ] [ Google Scholar ]
- 78. Mangabeira P.A., Ferreira A.S., De Almeida A.A.F., Fernandes V.F., Lucena E., Souza V.L., Dos Santos Júnior A.J., Oliveira A.H., Grenier-Loustalot M.F., Barbier F., Silva D.C. Compartmentalization and ultrastructural alterations induced by chromium in aquatic macrophytes. Biometals. 2011;24:1017–1026. doi: 10.1007/s10534-011-9459-9. [ DOI ] [ PubMed ] [ Google Scholar ]
- 79. Shanker A.K., Djanaguiraman M., Sudhagar R., Chandrashekar C.N., Pathmanabhan G. Differential antioxidative response of ascorbate glutathione pathway enzymes and metabolites to chromium speciation stress in green gram (Vigna radiata (L.) R.Wilczek. cv CO 4) roots. Plant Sci. 2004;166:1035–1043. doi: 10.1016/j.plantsci.2003.12.015. [ DOI ] [ Google Scholar ]
- 80. Pasricha S., Mathur V., Garg A., Lenka S., Verma K., Agarwal S. Molecular mechanisms underlying heavy metal uptake, translocation and tolerance in hyperaccumulators-an analysis: heavy metal tolerance in hyperaccumulators. Environ. Challenges. 2021;4 doi: 10.1016/j.envc.2021.100197. [ DOI ] [ Google Scholar ]
- 81. Park J.H. Contrasting effects of Cr(III) and Cr(VI) on lettuce grown in hydroponics and soil: chromium and manganese speciation. Environ. Pollut. 2020;266 doi: 10.1016/j.envpol.2020.115073. [ DOI ] [ PubMed ] [ Google Scholar ]
- 82. Hocaoglu-Ozyigit A., Gene B.N. Cadmium in plants, humans and the environment. Front. Life Sci. Relat. Technol. 2020;1:12–21. https://dergipark.org.tr/en/pub/flsrt/issue/56859/781913 [ Google Scholar ]
- 83. Sintorini M.M., Widyatmoko H., Sinaga E., Aliyah N. Effect of pH on metal mobility in the soil. IOP Conf. Ser. Earth Environ. Sci. 2021;737:6–12. doi: 10.1088/1755-1315/737/1/012071. [ DOI ] [ Google Scholar ]
- 84. Kubier A., Wilkin R.T., Pichler T. Cadmium in soils and groundwater: a review. Appl. Geochem. 2019;108 doi: 10.1016/j.apgeochem.2019.104388. [ DOI ] [ PMC free article ] [ PubMed ] [ Google Scholar ]
Associated Data
This section collects any data citations, data availability statements, or supplementary materials included in this article.
Data Availability Statement
- View on publisher site
- PDF (11.3 MB)
- Collections
Similar articles
Cited by other articles, links to ncbi databases.
- Download .nbib .nbib
- Format: AMA APA MLA NLM
Add to Collections
Advertisement
Experimental study on treatment of heavy metal–contaminated soil by manganese-oxidizing bacteria
- Research Article
- Published: 23 August 2021
- Volume 29 , pages 5526–5540, ( 2022 )
Cite this article
- Mengbo Liu 1 ,
- Shengli Wang 1 ,
- Meng Yang 1 ,
- Xiang Ning 1 &
- Zhongren Nan 1
1343 Accesses
13 Citations
1 Altmetric
Explore all metrics
There are many studies on the treatment of heavy metals by manganese-oxidizing bacteria and the reaction is good; the problem of compound pollution of heavy metals in soil has been difficult to solve. In this study, the application of manganese-oxidizing bacteria in soil was studied. The tolerance of manganese-oxidizing strains ( Pseudomonas taiwanensis ) to environmental conditions and the treatment effect of heavy metals As, Pb, and Cd in aqueous solution were investigated, and the effect of iron-manganese ratio on the treatment effect was discussed. The results showed that the suitable pH conditions for the growth of P. taiwanensis were 5–9, and the salt tolerance was 6% (by sodium chloride). The tolerant concentrations for heavy metals As(V) and Mn(II) were 500 mg L −1 and 120 mg L −1 , respectively. The strains were enriched by nutrient broth medium. After the logarithmic phase, the bacterial suspension was mixed with ATCC#279 medium at a ratio of 1:10, and a certain amount (10 mg L −1 ) of Mn(II) was added. The results of As, Pb, and Cd removal in the composite polluted water phase were 22.09%, 30.75%, and 35.33%, respectively. The molar ratio of manganese and iron affected the removal efficiency of single arsenic, the highest efficiency is 68%, and the ratio of iron to manganese is 1:5. However, when the soil was treated by the same method, the results showed that not all metals were passivated, such as Cu. At the same time, for As, Pb, and Cd, the treatment effects in soil were worse than those in water, perhaps more consideration should be given to environmental conditions, such as soil moisture and temperature, when manganese-oxidizing bacteria are used to treat soil.
This is a preview of subscription content, log in via an institution to check access.
Access this article
Subscribe and save.
- Get 10 units per month
- Download Article/Chapter or eBook
- 1 Unit = 1 Article or 1 Chapter
- Cancel anytime
Price excludes VAT (USA) Tax calculation will be finalised during checkout.
Instant access to the full article PDF.
Rent this article via DeepDyve
Institutional subscriptions
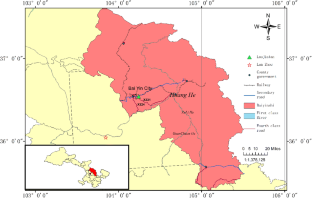
Similar content being viewed by others
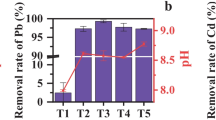
Combined treatment of heavy metals in water and soil by biochar and manganese-oxidizing bacteria

Enhancing Zn and Cd removal from heavy metal-contaminated paddy soil using an artificial microbial consortium
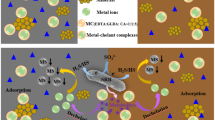
Enhanced biological stabilization of metal-chelant complexes in the chelator-washed soils by sulfate-reducing bacteria
Explore related subjects.
- Environmental Chemistry
Data availability
The datasets used or analyzed during the current study are available from the corresponding author on reasonable request.
Akob DM, Bohu T, Beyer A, Schäffner F, Händel M, Johnson CA, Merten D, Büchel G, Totsche KU, Küsel K, Lovell CR (2014) Identification of Mn(II)-oxidizing bacteria from a low-pH contaminated former uranium mine. Appl Environ Microbiol 80(16):5086. https://doi.org/10.1128/AEM.01296-14
Article CAS Google Scholar
Bai Y, Yang T, Liang J, Qu J (2016) The role of biogenic Fe-Mn oxides formed in situ for arsenic oxidation and adsorption in aquatic ecosystems. Water Res 98:119–127. https://doi.org/10.1016/j.watres.2016.03.068
Bai Y, Jefferson W, Liang J, Yang T, Qu J, Bai Y, Jefferson WA, Liang J, Yang T, Qu J (2017) Antimony oxidation and adsorption by in-situ formed biogenic Mn oxide and Fe-Mn oxides. J Environ Sci 54:126–134. https://doi.org/10.1016/j.jes.2016.05.026
Cao X, Ma LQ, Shiralipour A (2003) Effects of compost and phosphate amendments on arsenic mobility in soils and arsenic uptake by the hyperaccumulator, Pteris vittata L. Environ Pollut 126(2):157–167. https://doi.org/10.1016/S0269-7491(03)00208-2
Cavalcanti luna MA, Vieira ER, Okada K, Campos-takaki GM, AED N (2015) Copper-induced adaptation, oxidative stress and its tolerance in Aspergillus niger Ucp1261. Electron J Biotechnol 18(6):418–427. https://doi.org/10.1016/j.ejbt.2015.09.006
Article Google Scholar
Chen X, Lu X, Liu H, Li J, Xiang W, Zhang R, Lu J (2017) Oxidation and mineralization of Mn2+ ions mediated by Pseudomonas putida: insights from an experimental study. Acta Geologica Sinica - English Edition 91(4):1276–1285. https://doi.org/10.1111/1755-6724.13361
Cömert S, Tepe O (2020) Production and characterization of biogenic manganese oxides by manganese-adapted Pseudomonas putida Nrrl B-14878. Null 37(8):753–763. https://doi.org/10.1080/01490451.2020.1770900
Cui Z, Zhang X, Yang H, Sun L (2017) Bioremediation of heavy metal pollution utilizing composite microbial agent of Mucor circinelloides, Actinomucor sp and Mortierella sp. J Environ Chem Eng 5(4):3616–3621. https://doi.org/10.1016/j.jece.2017.07.021
Dong B, Zhang R, Gan Y, Cai L, Freidenreich A, Wang K, Guo T, Wang H (2019) Multiple methods for the identification of heavy metal sources in cropland soils from a resource-based region. Sci Total Environ 651:3127–3138. https://doi.org/10.1016/j.scitotenv.2018.10.130
Dong G, Han R, Pan Y, Zhang C, Liu Y, Wang H, Ji X, Dahlgren RA, Shang X, Chen Z, Zhang M (2021) Role of MnO2 in controlling iron and arsenic mobilization from illuminated flooded arsenic-enriched soils. J Hazard Mater 401:123362. https://doi.org/10.1016/j.jhazmat.2020.123362
Emerson D, Fleming EJ, Mcbeth JM (2010) Iron-oxidizing bacteria: an environmental and genomic perspective. Annu Rev Microbiol 64(1):561–583. https://doi.org/10.1146/annurev.micro.112408.134208
Fan D, Sun J, Liu C, Wang S, Han J, Agathokleous E, Zhu Y (2021) Measurement and modeling of hormesis in soil bacteria and fungi under single and combined treatments of Cd and Pb. Sci Total Environ 783:147494. https://doi.org/10.1016/j.scitotenv.2021.147494
Feng Q, Honbu C, Yanagisawa K, Yamasaki N (1999) Hydrothermal soft chemical reaction for formation of sandwich layered manganese oxide. Chem Mater 11(9):2444–2450. https://doi.org/10.1021/cm990133n
Gao P, Zeng X, Bai L, Wang Y, Wu C, Duan R, Su S (2017) As(v) Resistance and reduction by bacteria and their performances in as removal from as-contaminated soils. Curr Microbiol 74(9):1108–1113. https://doi.org/10.1007/s00284-017-1293-z
Haider FU, Coulter JA, Cheema SA, Farooq M, Wu J, Zhang R, Shuaijie G, Liqun C (2021) Co-application of biochar and microorganisms improves soybean performance and remediate cadmium-contaminated soil. Ecotoxicol Environ Saf 214:112112. https://doi.org/10.1016/j.ecoenv.2021.112112
Halan B, Vassilev I, Lang K, Schmid A, Buehler K (2017) Growth of Pseudomonas taiwanensis Vlb120∆c biofilms in the presence of n-butanol. Microb Biotechnol 10(4):745–755. https://doi.org/10.1111/1751-7915.12413
He Z, Zhu Y, Xu X, Wei Z, Wang Y, Zhang D, Pan X (2020) Complex effects of pH and organic shocks on arsenic oxidation and removal by manganese-oxidizing aerobic granular sludge in sequencing batch reactors. Chemosphere 260:127621. https://doi.org/10.1016/j.chemosphere.2020.127621
He B, Wang W, Geng R, Ding Z, Luo D, Qiu J, Zheng G, Fan Q (2021) Exploring the fate of heavy metals from mining and smelting activities in soil-crop system in Baiyin, Nw China. Ecotoxicol Environ Saf 207:111234. https://doi.org/10.1016/j.ecoenv.2020.111234
Holguera JG, Etui ID, Jensen LHS, Peña J (2018) Contaminant loading and competitive access of Pb, Zn and Mn(III) to vacancy sites in biogenic MnO2. Chem Geol 502:76–87. https://doi.org/10.1016/j.chemgeo.2018.10.020
Hou D, Zhang P, Wei D, Zhang J, Yan B, Cao L, Zhou Y, Luo L (2020) Simultaneous removal of iron and manganese from acid mine drainage by acclimated bacteria. J Hazard Mater 396:122631. https://doi.org/10.1016/j.jhazmat.2020.122631
Kang L, Zhang M, Liu Z, Ooi K (2007) IR spectra of manganese oxides with either layered or tunnel structures. Spectrochim Acta A Mol Biomol Spectrosc 67(3):864–869. https://doi.org/10.1016/j.saa.2006.09.001
Li J, Zhang P, Ye J, Zhang G, Cai Y (2019a) Simultaneous in-situ remediation and fertilization of Cd-contaminated weak-alkaline farmland for wheat production. J Environ Manag 250:109528. https://doi.org/10.1016/j.jenvman.2019.109528
Li LL, Yang J, Sun YJ, Yang XY, Chen RB, Zuo ZY, Yang ZH (2019b) Isolation and identification of Pseudomonas taiwanensis and its solubility in insoluble phosphate. J Wuhan Univ Sci Technol 42(5):354–364 (in Chinese)
Google Scholar
Li Y, Wang S, Nan Z, Zang F, Sun H, Zhang Q, Huang W, Bao L (2019c) Accumulation, fractionation and health risk assessment of fluoride and heavy metals in soil-crop systems in northwest China. Sci Total Environ 663:307–314. https://doi.org/10.1016/j.scitotenv.2019.01.257
Liu WJ (2012) Investigation and countermeasures of soil pollution around sewage irrigation area in Baiyin City. Gansu Sci Technol 28(v.28):28-29, 88 (in Chinese)
Liu J, Yao J, Duran R, Mihucz VG, Hudson-edwards KA (2019) Bacterial shifts during in-situ mineralization bio-treatment to non-ferrous metal(loid) tailings. Environ Pollut 255:113165. https://doi.org/10.1016/j.envpol.2019.113165
Lu N (2019) Research progress on the effects of combined pollution of Cd and Pb on physiology, biochemistry and cell structure of plants. Modern Agric Sci Technol 742(8):176–177 (in Chinese)
Manning BA, Fendorf SE, Bostick B, Suarez DL (2002) Arsenic(III) oxidation and arsenic(V) adsorption reactions on synthetic birnessite. Environ Sci Technol 36(5):976–981. https://doi.org/10.1021/es0110170
Matsushita S, Komizo D, Cao LTT, Aoi Y, Kindaichi T, Ozaki N, Imachi H, Ohashi A (2018) Production of biogenic manganese oxides coupled with methane oxidation in a bioreactor for removing metals from wastewater. Water Res 130:224–233. https://doi.org/10.1016/j.watres.2017.11.063
Meng Y, Zheng Y, Zhang L, He J (2009) Biogenic Mn oxides for effective adsorption of Cd from aquatic environment. Environ Pollut 157(8):2577–2583. https://doi.org/10.1016/j.envpol.2009.02.035
Moura H, Unterlass M, Moura HM, Unterlass MM (2020) Biogenic metal oxides. Biomimetics 5(2). https://doi.org/10.3390/biomimetics5020029
Nelson YM, Lion LW, Shuler ML, Ghiorse WC (2002) Effect of oxide formation mechanisms on lead adsorption by biogenic manganese (hydr)oxides, iron (hydr)oxides, and their mixtures. Environ Sci Technol 36(3):421–425. https://doi.org/10.1021/es010907c
Pacini VA, María ingallinella A, Sanguinetti G (2005) Removal of iron and manganese using biological roughing up flow filtration technology. Water Res 39(18):4463-4475. https://doi.org/10.1016/j.watres.2005.08.027
Qin W, Wei Q, Jiao F, Li N, Wang P, Ke L (2012) Effect of sodium pyrophosphate on the flotation separation of chalcopyrite from galena. Int J Min Sci Technol 22(3):345-349. CNKI:SUN:ZHKD.0.2012-03-012
Raja CE, Anbazhagan K, Selvam GS (2006) Isolation and characterization of a metal-resistant Pseudomonas aeruginosa strain. World J Microbiol Biotechnol 22(6):577–585. https://doi.org/10.1007/s11274-005-9074-4
Rebolledo UA, Páez-osuna F, Fernández R (2021) Single and mixture toxicity of As, Cd, Cr, Cu, Fe, Hg, Ni, Pb, and Zn to the rotifer Proales similis under different salinities. Environ Pollut 271:116357. https://doi.org/10.1016/j.envpol.2020.116357
Roberts TL (2014) Cadmium and phosphorous fertilizers: the issues and the science. Proc Eng 83:52–59. https://doi.org/10.1016/j.proeng.2014.09.012
Rousk J, Bååth E, Brookes PC, Lauber CL, Lozupone C, Caporaso JG, Knight R, Fierer N (2010) Soil bacterial and fungal communities across a pH gradient in an arable soil. ISME J 4(10):1340–1351. https://doi.org/10.1038/ismej.2010.58
Satapute P, Paidi MK, Kurjogi M, Jogaiah S (2019) Physiological adaptation and spectral annotation of arsenic and cadmium heavy metal-resistant and susceptible strain Pseudomonas taiwanensis. Environ Pollut 251:555–563. https://doi.org/10.1016/j.envpol.2019.05.054
Serrano S, O’day PA, Vlassopoulos D, García-gonzález MT, Garrido F (2009) A surface complexation and ion exchange model of Pb and Cd competitive sorption on natural soils. Geochim Cosmochim Acta 73(3):543–558. https://doi.org/10.1016/j.gca.2008.11.018
State Administration of Market Supervision, Ministry of Ecological Environment (2018) Standard for risk control of soil pollution in agricultural land for soil environmental quality ( Trial ). GB15618-2018, China
Tani Y, Miyata N, Ohashi M, Ohnuki T, Seyama H, Iwahori K, Soma M (2004) Interaction of inorganic arsenic with biogenic manganese oxide produced by a Mn-oxidizing fungus, strain Kr21-2. Environ Sci Technol 38(24):6618–6624. https://doi.org/10.1021/es049226i
Tessier A, Campbell PGC, Bisson M (1979) Sequential extraction procedure for the speciation of particulate trace metals. Anal Chem 51(7):844–851. https://doi.org/10.1021/ac50043a017
Villalobos M, Bargar J, Sposito G (2005) Mechanisms of Pb(II) sorption on a biogenic manganese oxide. Environ Sci Technol 39(2):569–576. https://doi.org/10.1021/es049434a
Wallace A (1982) Additive, protective, and synergistic effects on plants with excess trace-elements. Soil Sci 133(5):319–323. https://doi.org/10.1097/00010694-198205000-00009
Wan W, Tan J, Wang Y, Qin Y, He H, Wu H, Zuo W, He D (2020a) Responses of the rhizosphere bacterial community in acidic crop soil to pH: changes in diversity, composition, interaction, and function. Sci Total Environ 700:134418. https://doi.org/10.1016/j.scitotenv.2019.134418
Wan W, Xing Y, Qin X, Li X, Liu S, Luo X, Huang Q, Chen W (2020b) A manganese-oxidizing bacterial consortium and its biogenic Mn oxides for dye decolorization and heavy metal adsorption. Chemosphere 253:126627. https://doi.org/10.1016/j.chemosphere.2020.126627
Wang W, Shao Z, Liu Y, Wang G (2009) Removal of multi-heavy metals using biogenic manganese oxides generated by a deep-sea sedimentary bacterium – Brachybacterium sp. strain Mn32. Microbiology 155(6):1989–1996. https://doi.org/10.1099/mic.0.024141-0
Wang L, Tai C, Wu Y, Chen Y, Lee F, Wang S (2010) Pseudomonas taiwanensis sp. nov., isolated from soil. Int J Syst Evol Microbiol 60:2094–2098. https://doi.org/10.1099/ijs.0.014779-0
Wang H, Zhang D, Mou S, Song W, Al-misned FA, Golam mortuza M, Pan X (2015) Simultaneous removal of tetracycline hydrochloride and As(III) using poorly-crystalline manganese dioxide. Chemosphere 136:102–110. https://doi.org/10.1016/j.chemosphere.2015.04.070
Wang J, Xie Z, Wei X, Chen M, Luo Y, Wang Y (2020a) An indigenous bacterium Bacillus Xzm for phosphate enhanced transformation and migration of arsenate. Sci Total Environ 719:137183. https://doi.org/10.1016/j.scitotenv.2020.137183
Wang M, Chen S, Zheng H, Li S, Chen L, Wang D (2020b) The responses of cadmium phytotoxicity in rice and the microbial community in contaminated paddy soils for the application of different long-term N fertilizers. Chemosphere 238:124700. https://doi.org/10.1016/j.chemosphere.2019.124700
Wang Y, Tsang YF, Wang H, Sun Y, Song Y, Pan X, Luo S (2020c) Effective stabilization of arsenic in contaminated soils with biogenic manganese oxide (BMO) materials. Environ Pollut 258:113481. https://doi.org/10.1016/j.envpol.2019.113481
Watanabe J, Tani Y, Miyata N, Seyama H, Mitsunobu S, Naitou H (2012) Concurrent sorption of As(v) and Mn(ii) during biogenic manganese oxide formation. Chem Geol 306-307:123–128. https://doi.org/10.1016/j.chemgeo.2012.03.004
Watanabe J, Tani Y, Chang J, Miyata N, Naitou H, Seyama H (2013) As(III) oxidation kinetics of biogenic manganese oxides formed by Acremonium strictum strain Kr21-2. Chem Geol 347:227–232. https://doi.org/10.1016/j.chemgeo.2013.03.012
Xiao A, Li WC, Ye Z (2020) Effects of Fe-oxidizing bacteria (FEOB) on iron plaque formation, as concentrations and speciation in rice (oryza Sativa L.). Ecotoxicol Environ Saf 190:110136. https://doi.org/10.1016/j.ecoenv.2019.110136
Yan DY, Lo IM (2012) Pyrophosphate coupling with chelant-enhanced soil flushing of field contaminated soils for heavy metal extraction. J Hazard Mater 199-200:51–57. https://doi.org/10.1016/j.jhazmat.2011.10.054
Yan W, Hamid N, Deng S, Jia P, Pei D (2020) Individual and combined toxicogenetic effects of microplastics and heavy metals (Cd, Pb, and Zn) perturb gut microbiota homeostasis and gonadal development in marine medaka (Oryzias melastigma). J Hazard Mater 397:122795. https://doi.org/10.1016/j.jhazmat.2020.122795
Zhang YJ, Guo SL (2019) Effects of environmental factors on soil microbial respiration and its temperature sensitivity. Environ Sci 40(3):1446–1456 (in Chinese)
Zhang Z, Yin H, Tan W, Koopal LK, Zheng L, Feng X, Liu F (2014) Zn sorption to biogenic bixbyite-like Mn2O3 produced by Bacillus cua isolated from soil: Xafs study with constraints on sorption mechanism. Chem Geol 389:82–90. https://doi.org/10.1016/j.chemgeo.2014.09.017
Zhang W, Wang C, Xue R, Wang L (2019a) Effects of salinity on the soil microbial community and soil fertility. J Integr Agric 18(6):1360–1368. https://doi.org/10.1016/S2095-3119(18)62077-5
Zhang Y, Tang Y, Qin Z, Luo P, Ma Z, Tan M, Kang H, Huang Z (2019b) A novel manganese oxidizing bacterium-Aeromonas hydrophila strain Ds02: Mn(II) oxidization and biogenic Mn oxides generation. J Hazard Mater 367:539–545. https://doi.org/10.1016/j.jhazmat.2019.01.012
Zhou D, Kim D, Ko S (2015) Heavy metal adsorption with biogenic manganese oxides generated by Pseudomonas putida strain Mnb1. J Ind Eng Chem 24:132–139. https://doi.org/10.1016/j.jiec.2014.09.020
Download references
Acknowledgements
We are very grateful to Xiangliang Pan Research Group of Zhejiang University of Technology for providing technical support.
In this study, economic support was provided by the National Key Research and Development Program of China (2018YFC1802905).
Author information
Authors and affiliations.
College of Earth and Environmental Sciences, Lanzhou University, 222 Tianshui South Road, Lanzhou, 730000, China
Mengbo Liu, Shengli Wang, Meng Yang, Xiang Ning & Zhongren Nan
You can also search for this author in PubMed Google Scholar
Contributions
All authors made an indispensable contribution to this study. The technical guidance and methods were provided by Shengli Wang. The writing and analysis of the article were completed by Mengbo Liu. The data processing and image production were carried out by Xiang Ning. The experimental scheme and operation were carried out by Meng Yang. The experimental materials and fonds were carried out by Zhongren Nan.
Corresponding author
Correspondence to Shengli Wang .
Ethics declarations
Ethics approval and consent to participate.
Not applicable.
Consent for publication
Competing interests.
The authors declare no competing interests.
Additional information
Responsible Editor: Diane Purchase
Publisher’s note
Springer Nature remains neutral with regard to jurisdictional claims in published maps and institutional affiliations.
Rights and permissions
Reprints and permissions
About this article
Liu, ., Wang, S., Yang, M. et al. Experimental study on treatment of heavy metal–contaminated soil by manganese-oxidizing bacteria. Environ Sci Pollut Res 29 , 5526–5540 (2022). https://doi.org/10.1007/s11356-021-15475-0
Download citation
Received : 01 April 2021
Accepted : 13 July 2021
Published : 23 August 2021
Issue Date : January 2022
DOI : https://doi.org/10.1007/s11356-021-15475-0
Share this article
Anyone you share the following link with will be able to read this content:
Sorry, a shareable link is not currently available for this article.
Provided by the Springer Nature SharedIt content-sharing initiative
- Soil pollution
- Heavy metal
- Microbial remediation
- Manganese-oxidizing bacteria
- Pseudomonas taiwanensis
- Biogenic manganese oxides
- Find a journal
- Publish with us
- Track your research

IMAGES
COMMENTS
Lead is called a heavy metal, and there are other sources of heavy metals that can be toxic, too. Silver, copper, mercury, nickel, cadmium, arsenic, and chromium are all heavy metals that can be toxic in certain environments. In this experiment, find out if one common heavy metal, copper, can be toxic to an aquatic environment.
Metal science fair projects and experiments: topics, ideas, resources, and sample projects by scientific field. ... See if heavy metal has negative effects, both ...
Dec 11, 2015 · A Heavy Metal Song – masaru-emoto.net While most music genres used in these experiments positively influence water and create such beautiful designs, Heavy Metal simply seems to put the water’s “inner energy” into a deep well of basically … shit.
Feb 15, 2022 · A series of laboratory experiments were performed to explore the efficiency and mechanism of S. pasteurii-based bioremediation to heavy metal contaminated solutions under various conditions, including single-heavy-metal pollution condition, heavy metal pollution under high mineral salinity context and multi-heavy-metal pollution scenarios.
Oct 20, 2020 · Mesocosms are real-world environmental science tools for bridging the gap between laboratory-scale experiments and actual habitat studies on ecosystem complexities. These experiments are increasingly being applied in understanding the complex impacts of heavy metals, ocean acidification, global warming, and oil spills. The insights of the present review indicate how metals and metal-bound ...
Dec 11, 2018 · These experiments are increasingly being applied in understanding the complex impacts of heavy metals, ocean acidification, global warming, and oil spills. The insights of the present review indicate how metals and metal-bound activities impact on various aspects of ecological complexities like prey predator cues, growth, embryonic development ...
Mesocosm experiments on the impact of heavy metals in marine environment Heavy metals are brought into the aquatic system from a number of natural and anthropogenic sources such as weathering of soil, rocks, volcanic eruptions, mining, processing of metals, industrial and urban effluents, and ports and harbor activities (Lin et al. 2012; El ...
Apr 4, 2024 · The pot experiment involving varying concentrations of Cd, Cr, and Pb served to systematically explore the responses of B. chinensis and B. rapa to different levels of heavy metal contamination. This approach established a dose-response relationship, allowing researchers to identify threshold levels impacting germination, growth rates, and ...
Aug 23, 2021 · Heavy metal removal experiment in water solution. First, we set (1) As, (2) Pb, (3) Cd, and (4) As + Pb + Cd four groups of experiments, and each set of blank control (without treatment). Taking group (1) as an example, manganese-oxidizing strains were first inoculated into nutrient broth medium and cultured in the logarithmic phase (14–16 h).
Sep 23, 2020 · Through filler selection experiment, the static adsorption and dynamic removal effects of N-HAP on heavy metal ions are the best, reaching 100%, far better than the other three fillers. The removal effect of N-HAP on Zn 2+ and Cu 2+ was not significantly changed compared with the first water injection, and the removal rate was still 100% ...